The mechanism and thermodynamics of ethyl alcohol sorption process on activated petroleum coke
- 1 — Ph.D., Dr.Sci. Professor Empress Catherine II Saint Petersburg Mining University ▪ Orcid
- 2 — Postgraduate Student Empress Catherine II Saint Petersburg Mining University ▪ Orcid
- 3 — Laboratory Technician Empress Catherine II Saint Petersburg Mining University ▪ Orcid
- 4 — Ph.D. Executive Director of Scientific Center “Issues of Processing Mineral and Technogenic Resources” Empress Catherine II Saint Petersburg Mining University ▪ Orcid
Abstract
The low-quality petcoke does not find qualified application and is stockpiled at refineries or used as solid fuel. One of the promising ways to use low-quality petroleum coke is its physical or chemical activation in order to obtain a highly porous carbon material that can be used as a catalyst carrier, adsorbent, base for electrodes, etc. The possibility of using petroleum coke to produce sorbent for organic compounds was studied. The activated petroleum cake was obtained by chemical activation with KOH, a specific surface area is 1218 m2/g. Sorption of ethyl alcohol was studied at temperatures 285, 293 and 300 K. It is a physical process proceeding mainly in pores of activated petroleum coke, also sorption can be described as a reversible exothermic process. The effective Gibbs energy at a temperature of 293 K is –12.74 kJ/mol, the heat of sorption is –26.07 kJ/mol. The obtained data confirm that porous carbon material obtained from petroleum coke can be used as sorbent for ethanol at room temperature. For example, for adsorption of bioethanol from the effluent of the fermentation process or for purification of wastewater from organic compounds.
Introduction
The sorption capacity depends on the surface structure of the material, so carbon materials with high specific surface area are better to use for sorption [1-3], for example, activated carbon. Activated carbons are obtained from various raw materials [4-6], however, it is ecologically and economically feasible to use waste products for the production of porous carbon material. Petroleum coke can be such a raw material because it is produced as a by-product of the delayed coking, which allows for deeper refining of crude oil. The process of delayed coking of oil residues is the most economically demanded among all the varieties of the thermal processes of crude oil deep processing. When high-sulphur feedstock is used, the obtained high-sulphur low-quality coke is not widely used and stored at the refinery or used as solid fuel. Such application causes environmental problems, so alternative ways of using petroleum coke must be found [7-9].
One of the promising uses of high-sulfur petroleum coke is its physical or chemical activation to produce a highly porous carbon material that can be used as a catalyst carrier or adsorbent. Activation of petroleum coke is carried out by mixing of carbon material with an activating agent (КОН, NaОН, ZnCl2 etc.) [10-12] or by gas treatment (СО2, water vapor etc.) [13]. Depending on the activating agent, carbon material with different pore structure and specific surface area can be produced. KOH develops microporosity, and the surface area varies from 1,700 to 2,500 m2/g depending on the ratio of KOH:coke (from 3:1 to 6:1) [14, 15]. In comparison with KOH, sodium hydroxide gives lower porosity and specific surface area 600-1,397 m2/g, but larger pore diameter [16-18]. A possible explanation for this can be found in Pearson's theory [19, 20]. Potassium and sodium ions are “hard” acids according to Pearson's classification, and the “hardness” of an acid is directly proportional to the ratio of the ion's charge to its radius. The “hardness” of sodium and potassium ions is 21.08 and 13.64 respectively [20]. Carbon is a “soft” base and, according to Pearson's theory, it will favorably react with “soft” acids. Since the “hardness” of potassium is lower than the “hardness” of sodium, the potassium-carbon interaction will be more favored, and hence the porous structure will be more developed as a result of chemical interactions [16, 21]. In addition, the hydroxide ion is a “hard” base according to Pearson, thus the more “hard” sodium is more strongly bound to the “hard” hydroxide ion, making it more difficult for the sodium hydroxide to dissociate in the melt. As a result, the chemical interaction between NaOH and petroleum coke is hindered, resulting in less development of porous structure compared to interaction with KOH over the same period of time.
Sodium and potassium carbonates poorly develop the porosity of petroleum coke at temperatures of 973-1,073 K [17, 22, 23]. A specific surface area of 1,000-2,000 m2/g was obtained by activation with zinc chloride. Physical activation is conducted with gases, usually steam or CO2, at temperatures of 973-1,173 K in an inert atmosphere for 6-9 hours. The specific surface area of the samples after activation is 400-800 m2/g [11]. Thus, KOH is the most promising activating agent for obtaining highly porous carbon material.
The ratio of carbon material to activating agent is also an important parameter for porous structure formation. When the ratio of carbon material:KOH increases, the specific surface area and pore volume also increase, but the yield of porous material decreases. Thus, when the petroleum coke was activated at 1,073 K, the specific surface area was 379; 845; 1,867; 1,940; 2,438 m2/g at the mass ratio of KOH:petroleum coke of 0.5; 1; 2; 3 and 5 respectively [14, 17, 24]. A ratio higher than 6 reduces the surface area of the activated material. A KOH:coke ratio from 3 to 5 is necessary to maximize the surface area [24].
The surface area and pore volume increase with increase in activation temperature, but only up to the temperature of 1,023-1,073 K. Further increase in temperature decreases the surface area of the sample, which may be due to the destruction of thin pore walls [14, 25, 26].
The obtained activated material can be used as sorbent for wastewater and gas purification, as catalyst carriers, for electric double-layer capacitors, for adsorption of bioethanol from the effluent of the fermentation process, etc. [27].
The process of sorption on carbon materials is complex, since many factors – specific surface area, pore size, surface homogeneity, etc. – will affect the kinetics and thermodynamics of the process [28].
The sorption capacity of the carbon material strongly depends on the specific surface area and porosity [27, 29, 30]. For two carbon materials with close values of specific surface area of 307 and 486 m2/g maximum sorption capacities for methanol, ethanol, n-propanol and n-butanol at temperature of 298 K are close. The highest sorption capacity was obtained for methanol – 3.7 mol/kg. The sorption capacity decreases with augmentation of the alkyl chain. When the specific surface area increases up to 3,023 m2/g the sorption capacity increases and is 16 mol/kg for methanol, and the trend of decreasing sorption capacity at transition from methanol to n-butanol persists [31]. Thus, alcohol sorption proceeds mainly in the pores of the carbon material, where the steric effect has a significant influence on the efficiency of the process.
The adsorption of polar molecules, such as alcohol, is closely related to the chemical composition of the adsorbent surface [28]. Surface oxygen groups (carboxyl, hydroxyl, lactone, etc.) have a great influence on the sorption process. The maximum sorption capacity does not depend on the presence of oxygen groups on the sorbent surface, but at low values of pressure or concentration alcohol molecules are mainly adsorbed on oxygen groups [31]. Thus, the sorption at low pressure increases with increasing number of oxygen functional groups.
The Langmuir, Temkin, Freundlich and Dubinin – Radushkevich models are most commonly used to calculate the thermodynamic parameters of the sorption of alcohols on carbon materials [3, 27]. The Langmuir model is based on the consideration of a monolayer of adsorbate on the adsorbent sur-face and does not take into account volumetric sorption in the activated coke micropores [32]. To describe adsorption in micropores, the Dubinin – Radushkevich model of volumetric filling of micropores was developed [33, 34]. The Freundlich model takes into account sorption on inhomogeneous surface. The coefficient n is a measure of the deviation of adsorption from linearity. If n < 1, adsorption modifies the sorbent in a way that increases the sorption capacity by forming new adsorption centers, this is a chemical sorption. However, if n > 1, adsorption bond becomes weak, and the sorption capacity changes significantly with small changes in concentration. In this case, physical adsorption takes place. Temkin model takes into account heterogeneity of the sorbent surface and adsorbate-adsorbate interaction, which takes place in the sorbent micropores. The mathematical representation of the models is shown in Table 1.
Table 1
Mathematical models to describe the thermodynamic equilibrium of sorption [35]
Model |
Nonlinear form |
Linear form |
Langmuir |
where q and q∞ – sorption capacity at the adsorbate concentration X∞ and maximum respectively, mol/kg; X∞ – molar ratio of the adsorbate at equilibrium; KL – Langmuir constant |
|
Freundlich |
where KF and n – Freundlich constants |
|
Temkin |
where R – universal gas constant (8.31 J/mol∙К); Т – temperature, K; KT and AT – Temkin constants |
|
Dubinin – Radushkevich |
where |
|
The Langmuir model better describe the sorption of alcohols on materials with low specific surface area. Ethanol sorption on carbon material with a specific surface area of 1,904 m2/g was badly described by the Langmuir model with a correlation coefficient of 0.45-0.73, whereas ethanol sorption on activated carbon with a specific surface area of 871 m2/g was well described by this model [27]. Also, the Langmuir model better describes the sorption process when the length of the alkyl radical of alcohols increases. The Langmuir model agrees well with experimental data for isopropyl alcohol sorption with a correlation coefficient of R2 = 0.96-0.999, and Freundlich model gives a good fit to the experimental data for ethanol sorption with correlation coefficient of R2 = 0.84-0.99 [1]. This dependence may be due to a decrease in the amount of sorption in the pores of the sorbent, compared to its surface, with a decrease in the surface area and an increase in the length of the alkyl chain of the alcohols.
The length of the alkyl radical of alcohols has a significant effect on the heat effect of the reaction. The enthalpy values of methanol adsorption on activated carbon range from 40 to 55 kJ/mol, and increasing the alkyl chain by one –CH2– increases the enthalpy of adsorption by approximately 5 kJ/mol [3, 36].
Many studies focus on the sorption of alcohol vapor on carbon materials, and only a few reports are devoted to the study of the sorption of alcohols from aqueous solution [27]. Moreover, the existing studies focus on coal or activated carbon as a sorbent, but do not consider activated material from technogenic feedstock – petroleum coke. Activated petroleum coke has a high specific surface area 1,200-2,500 m2/g [37-39] and can contain oxygen functional groups [21, 40], which makes it a promising sorbent for alcohol sorption.
The article presents a study of ethyl alcohol sorption on activated petroleum coke with high specific surface area 1,200 m2/g to determine the possibility of its application as a sorbent for ethyl alcohol from aqueous solutions. This material can be used for adsorption of bioethanol from the effluent of the fermentation process which is a promising technique for production of fuel-grade bioethanol. Implementation of the research results will solve two environmental problems – low-quality petroleum coke will be used and the production of fuel-grade bioethanol will be organized.
Methods
The research was carried out in the Laboratory of Innovative Refining Technologies of the Scientific Center “Issues of Mineral and Technogenic Resources Processing” of the Empress Catherine II Saint Petersburg Mining University.
Petroleum coke was obtained from vacuum residue in the laboratory delayed coking unit at a coking temperature of 773 K and overpressure of 1.5-3.5 atm. The delayed coking process and petroleum coke characteristics are described in more details in the article [41].
Activation of petroleum coke was carried out in a 100 ml porcelain crucible in a furnace Nabertherm LHT 08/17 with mass ratio of KOH to petroleum coke equal 3. The mixture was heated to 1,023 K at a heating rate of 10 K/min in N2 medium at a flow rate of 100 ml/min. The samples were kept at the final temperature for 1 h and then cooled to room temperature in N2 flow. Washing was carried out first with 1 mol/L HCl, then with distilled water until the pH of the wash water was neutral. The obtained samples were dried at temperate of 393 K for 3 h. Optimal activation parameters for obtaining highly porous carbon material were selected based on literature data, activated petroleum coke characteristics are described in more detail in article [41].
Determination of specific surface area and pore size distribution for activated coke was carried out by the BET method on a device Quantachrome NOVA3200e. Before analysis the samples were degassed under vacuum at temperate of 473 K for 6 h. The specific surface area of the obtained material was 1,219 m2/g.
Nitrogen adsorption isotherm for activated carbon (Fig.1, a) corresponds to type I, typical for microporous materials [22]. The linear form of nitrogen adsorption isotherm for activated carbon is shown in Fig.1, b.
Table 2
Pore size distribution in activated petroleum coke, %
Microporous> 2 nm |
Mesoporous |
Macroporous50-100 nm |
|
2-5 nm |
5-50 nm |
||
62.46 |
30.42 |
5.90 |
1.22 |
The pore size distribution is shown in Table 2. The obtained values agree with the data reported in the literature [38].
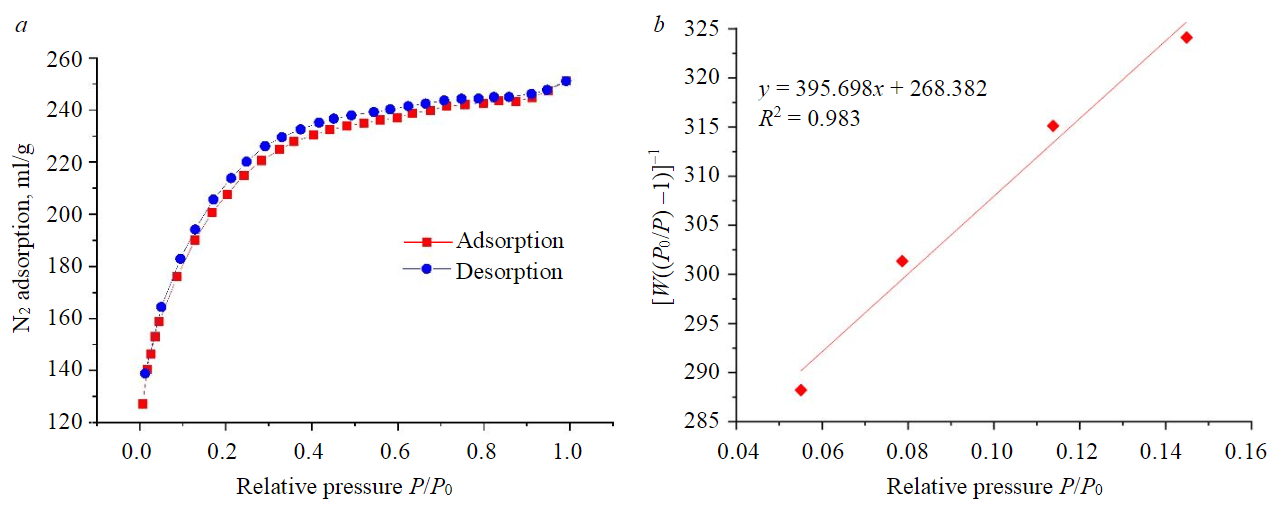
Fig.1. Nitrogen adsorption isotherm (a) and linear form of the isotherm according to the BET model (b)
IR spectra of coke were obtained on a Shimadzu IRAffinity-1 Fourier infrared spectrometer. For analysis, 5 mg of petroleum coke was mixed with 500 mg of KBr and stirred in a mortar until homogeneous mixture was obtained. Then 300 mg of the mixture was taken and pressed at 5.5 MPa. All spectra were taken relative to KBr.
IR spectra of activated coke are shown in Fig.2. The absorption bands in the 3,500-3,400 cm–1 are related to the stretch vibration of the ОН-groups, which determine the moisture content in the sample. Peak at 781 cm–1 is related to С–Н deformation vibrations. The broad peak at 1,400-1,160 cm–1 is responsible for the sulfur-containing compounds and deformation vibrations of the С–Н-groups. Thus, no oxygen-containing compounds, such as organic acids or esters that could be functional surface groups, were found in the activated petroleum coke. However, the broad peak at 930-520 cm–1 may be related to the superposition of the peak of CO2-3 valent vibrations on the peak of C-H deformation vibrations, formed as a result of the activation of petroleum coke by KOH.
The elemental composition of petroleum coke was obtained using carbon, hydrogen, nitrogen analyzer LECO CHN628 and X-ray fluorescence spectrometer EDX-7000P Shimadzu [41]: 81.7 % carbon, 13.3 % oxygen, 1.84 % hydrogen, 2 % potassium. The content of other elements in activated petroleum coke is less than 1 %.
The composition of activated petroleum coke, %: 81.71 С; 13.32 O; 1.84 Н; 0.61 N; 0.50 S; 2.03 K; 0.03 Fe.
Sorption of ethyl alcohol was carried out in a shaker-thermostat at an alcohol solution:coke ratio of 30:1 at a temperature of 285 to 300 K with a stirring speed of 20 to 150 rpm for two hours. The concentration of ethyl alcohol in the aqueous solution was changed from 2.5 to 40 vol.%.
Alcohol concentration in the initial solution and in the solution after sorption was determined by gas chromatography on the chromatograph “Kristall-5000” by the method of internal standard. Propanol-1 was used as an internal standard.
The sorption capacity of activated petroleum coke was calculated by the equation
where С0 – initial concentration of alcohol in aqueous solution, mol/L; С∞ – equilibrium concentration of alcohol in aqueous solution, mol/L; V – volume of the solution taken for sorption, ml; m – mass of the sorbent, g.
Gibbs energy is calculated by the equation
where Kр – equilibrium constant of the adsorption process.
The enthalpy of sorption ΔH is determined by the isobar equation
In a narrow temperature range (a few tens of degrees) heat effect of a chemical reaction ΔH is independent of temperature. Therefore, after integrating equation (1) we obtain
where А – the integration constant, equal to
According to equation (2), the slope of the dependence lnKp vs. T–1 is equal to
Activities of ethyl alcohol in the aqueous phase are calculated by the equation
where xA – molar ratio; γA – rational activity coefficient.
Activity coefficients γA were determined by a modified model UNIFAC, which well describes aqueous solution of alcohols. The calculation was made using the software Pytherm*.
The molecular mass of coke is calculated by equation
where ωi – mass ratio of an element in the petroleum coke, %; Mi – molecular mass of an element, g/mol.
The molecular mass of the carbon material is used to calculate the molar ratio of ethyl alcohol in the “sorbent” phase
where νA – amount of ethanol sorbed on activated petroleum coke, mol.
Discussion
Isotherms of ethyl alcohol sorption on activated petroleum coke at different temperatures and the effect of stirring speed on the sorption process are presented in Fig.3.
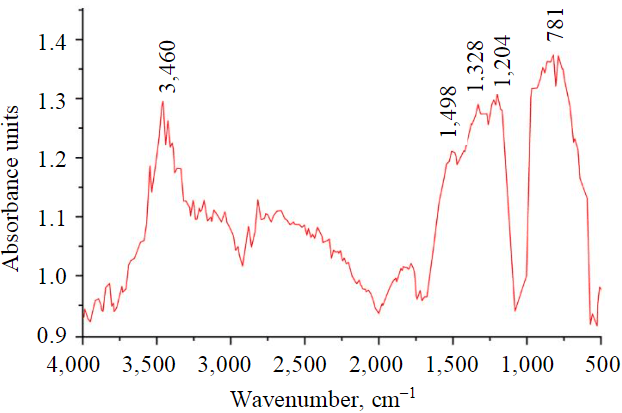
Fig.2. IR spectra of activated petroleum coke
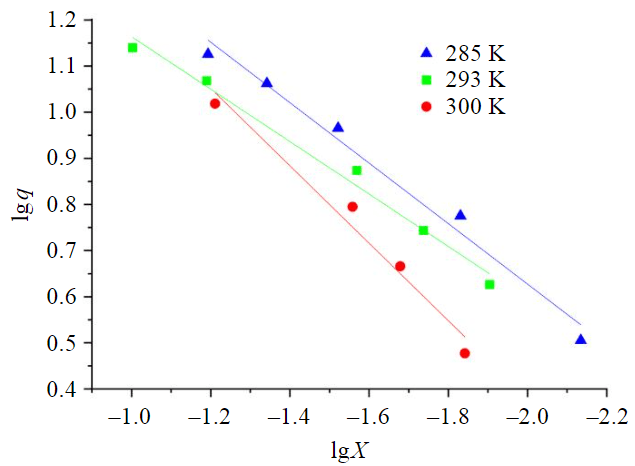
Fig.4. Linear form of the Freundlich adsorption isotherm
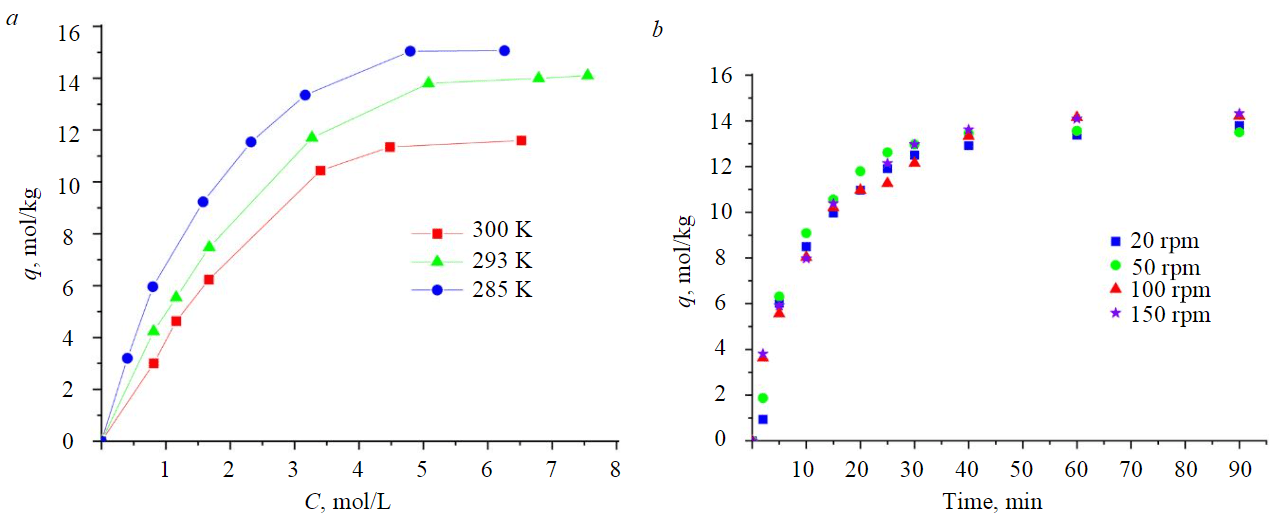
Fig.3. Sorption of ethyl alcohol on activated petroleum coke at 285, 293 and 300 K (а) and influence of stirring speed on the sorption process at 293 K(b)
In the investigated range of ethyl alcohol concentrations, the isotherm form corresponds to monomolecular adsorption for all three temperatures. The maximum capacity of the sorbent at the studied temperatures is 15.0, 14.1 и 11.6 mol/kg for temperatures 285, 293 and 300 K respectively. The decrease in sorption capacity with increasing temperature indicates the exothermic process, which is typical for physical sorption. The obtained values of maximum sorption capacity are higher than the values presented in the literature [27, 42] for activated carbons 3-5 mol/kg, i.e. this material removes alcohol from aqueous solution more efficiently than industrial activated carbons.
The absence of dependence of ethyl alcohol sorption on the stirring speed (Fig.3, b) shows that sorption predominantly takes place on the internal surface or in the pores of the activated coke. This is facilitated by the developed surface of the material and the large number of micropores larger than 0.4 nm in which an ethanol molecule (d = 0.4 nm) can sorb.
Two parts can be found on the isotherm (Fig.3), corresponding to linear and nonlinear dependence of sorption on the concentration of the sorbate. Deviation from linearity can be associated with a decrease in the free surface area and the sorption in the pore.
The Freundlich model was used to establish the character of sorption (Fig.4), which well enough describes the experimental data. The linear approximation equations are shown in Table 3.
Table 3
Freundlich equation for temperatures 285, 293 and 300 K
T, K |
The approximation equation |
R2 |
KF |
n |
285 |
|
0.98 |
86 |
1.53 |
293 |
|
0.99 |
53 |
1.76 |
300 |
|
0.95 |
49 |
1.69 |
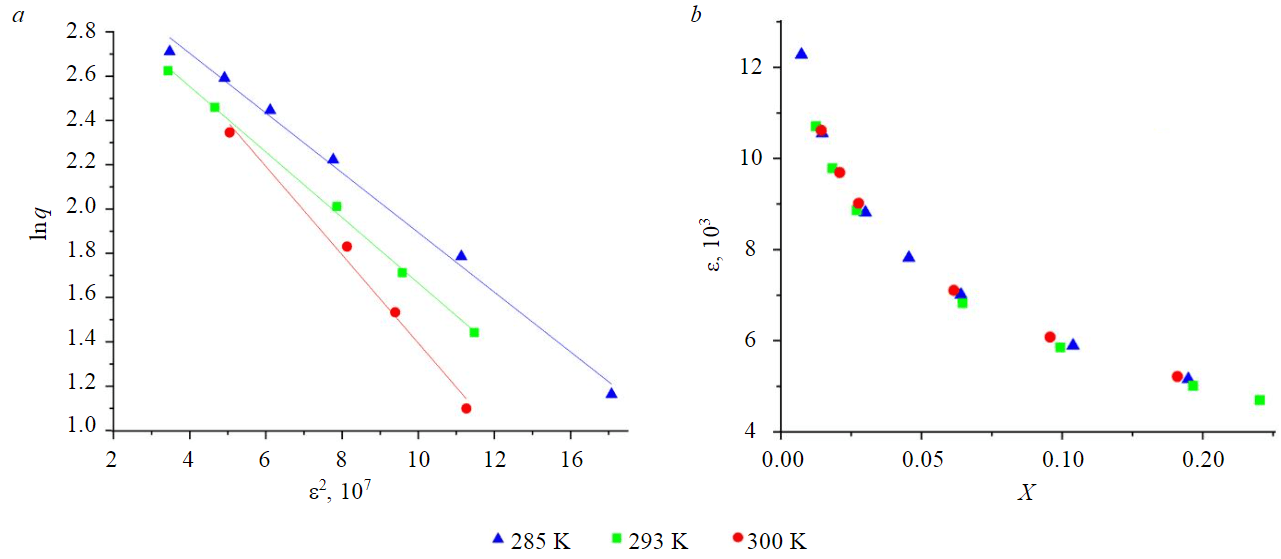
Fig.5. Linear form of the Dubinin – Radushkevich adsorption isotherm (a) and dependence of the coefficient e on temperature (b)
The value of the coefficient n > 1 indicates the physical character of alcohol adsorption on the surface of activated petroleum coke. Freundlich constant decreases with increasing temperature, which is typical for molecular adsorption.
The participation of micropores in the sorption process is confirmed by a good approximation of the experimental data by the Dubinin – Radushkevich model (Fig.5). The value of the coefficient ε does not depend on the temperature, is determined by the equilibrium concentration of the alcohol and varies from 4.69 to 12.28 kJ/mol. The Dubinin – Radushkevich model well fits the experimental data on the nonlinear part of the sorption isotherm (Table 4).
The correlation coefficient at 300 K for the Dubinin – Radushkevich model increases compared to the Freundlich model, indicating that the contribution of micropores to the sorption process increases with increasing temperature. The value of maximum sorption decreases with increasing temperature, which is typical for molecular sorption. The average adsorption free energy E is 5.6-6.1 kJ/mol (which is less than 8 kJ/mol), also shows that physical rather than chemical sorption is taking place.
The Dubinin – Radushkevich model ignores the influence of the solvent on chemical transformations of dissolved substances, as well as the surface charge and dissociation of functional groups of the sorbent in the sorption system solid – solution [33]. Thus, the Dubinin – Radushkevich model does not fully describe the sorption interaction.
Table 4
Dubinin – Radushkevich equation for temperatures 285, 293 and 300 K
T, K |
The approximation equation |
R2 |
KDR∙107 |
E, kJ/mol |
q∞ |
285 |
|
0.99 |
0.14 |
6.08 |
25.0 |
293 |
|
0.99 |
0.15 |
5.81 |
22.7 |
300 |
|
0.98 |
0.16 |
5.61 |
21.3 |
The Freundlich and Dubinin – Radushkevich models are poorly informative for establishing thermodynamic characteristics of sorption. The sorption equilibrium constant, heat of adsorption and Gibbs energy are preferably calculated using the Langmuir model in the case of monomolecular sorption on the surface of the sorbent or the Temkin model if sorption in the pores of the sorbent is assumed.
In the range of concentrations corresponding to the linear part of the isotherm, sorption of alcohol on the surface of activated petroleum coke at low degrees of surface filling can be described by the quasi-chemical equation
where А and
Then the effective equilibrium constant of the process can be written as follows:
where
Equation (3) lets calculate the equilibrium constant at the first linear section of the sorption isotherm, where Henry's law is satisfied. The calculated equilibrium constants Keff of ethyl alcohol sorption on activated petroleum coke at different temperatures are presented in Table 5.
Table 5
Equilibrium constants of the sorption process, calculated according to the law of mass action
Т, K |
|
аA |
Keff |
△G, kJ/mol |
285 |
0.040 |
0.032 |
1.27 |
–0.56 |
293 |
0.052 |
0.055 |
0.96 |
0.11 |
300 |
0.038 |
0.065 |
0.58 |
1.35 |
The sorption equilibrium constant decreases with increasing temperature, which is confirmed by the conclusion about the exothermicity of the process. The low value of the equilibrium constant and the Gibbs energy value of –0.56 to 1.35 kJ/mol indicate the reversibility of the process.
Values of equilibrium constants (Table 5) were used to calculate the enthalpy of the adsorption process using equation (2) by plotting the linear dependence of lnKp vs. T–1. The correlation coefficient for the approximation equation
Application of the Langmuir model taking into account the non-ideality of the sorbent solution is possible at its concentrations slightly exceeding the Henry region (Fig.6). The effective values of the equilibrium constant and Gibbs energy were obtained from Langmuir isotherms plotted taking into account the non-ideality of the solution (Table 6).
An increase in temperature leads to a deterioration in the applicability of the Langmuir isotherm, which is apparently due to increased sorption in the pores of activated petroleum coke and agrees with the data obtained from the analysis of the Dubinin – Radushkevich isotherm.
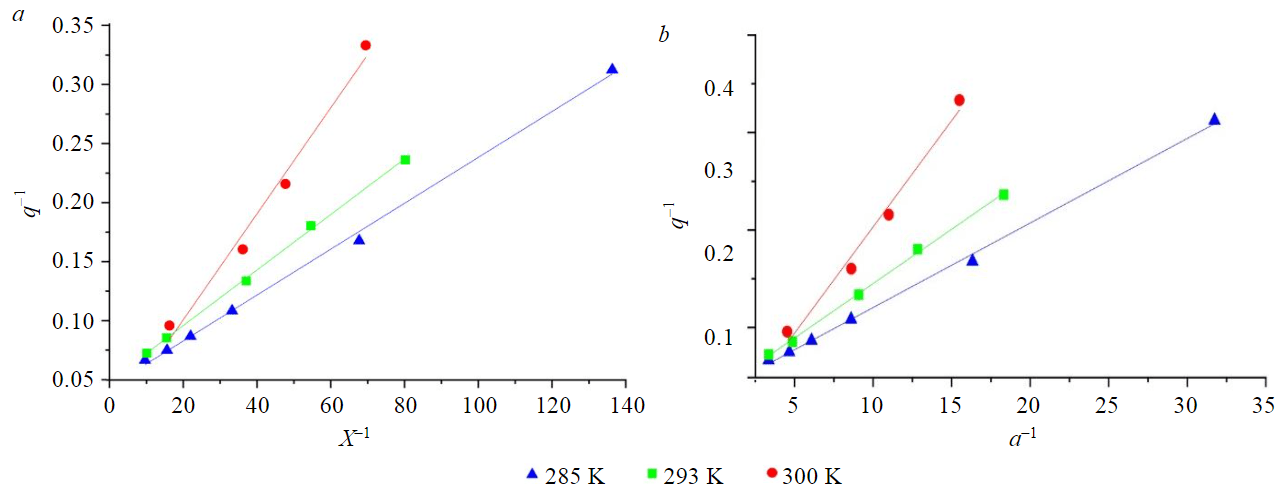
Fig.6. Langmuir isotherms plotted for concentrations (а) and activities (b)
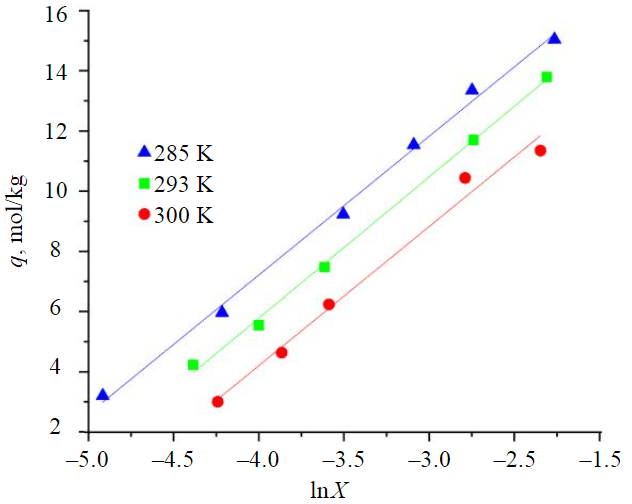
Fig.7. Linear form of the Temkin adsorption isotherm
The experimentally obtained maximum sorption capacity decreases with increasing temperature and is 15.0, 14.1 and 11.5 mol/kg for 285, 293 and 300 K respectively. However, according to the Langmuir model, the maximum sorption capacity increases from 29.60 to 67.43 mol/kg with an increase in temperature from 285 to 300 K, which contradicts the dependence obtained experimentally – the decrease in the value of the maximum sorption with increasing temperature. This is due to the fact that the Langmuir model does not take into account volumetric sorption in the micropores of activated coke and considers only a monolayer of adsorbate on the adsorbent surface.
The highest correlation coefficient for all three temperatures is given by the Temkin model (Fig.7), which not only takes into account the heterogeneity of the sorbent surface, but also the adsorbate-adsorbate interaction that takes place in the sorbent micropores.
The values of equilibrium constants KT and Gibbs energies calculated by the Temkin model are presented in Table 7.
The lower Gibbs energy values obtained by the Temkin model can be related both to the predominance of sorption in the pores as the alcohol concentration increases and to the fact that the Temkin model was obtained in the ideal solution approximation.
Values of equilibrium constants (Table 7) were used to calculate the enthalpy of the adsorption process using equation (2), by plotting the linear dependence of lnKp vs. T–1. The correlation coefficient is 0.997 for the approximation equation
The negative value of reaction enthalpy indicates that the process is exothermic and low Gibbs energy of –13 to –12 kJ/mol – that the process runs near the equilibrium.
Table 6
Langmuir equations for temperatures 285, 293 and 300 K
T, K |
The approximation equation |
R2 |
KL |
q∞, mol/kg |
△G, kJ/mol |
285 |
|
0.99 |
3.90 |
29.60 |
–3.22 |
293 |
|
0.99 |
3.00 |
29.85 |
–2.68 |
300 |
|
0.98 |
0.68 |
67.43 |
0.96 |
Table 7
Temkin equation for temperatures 285, 293 and 300 K
T, K |
The approximation equation |
R2 |
KT |
△G, kJ/mol |
285 |
|
0.99 |
260 |
–13.18 |
293 |
|
0.99 |
187 |
–12.74 |
300 |
|
0.99 |
135 |
–12.24 |
The law of mass action describes only the initial part of sorption, where Henry’s law works, whereas to describe the sorption process as a whole it is better to use the values obtained by the Temkin model.
Therefore, the process of sorption of ethanol on activated petroleum coke is a physical interaction and involves both a heterogeneous surface and micropores of the sorbent. There is no polymolecular sorption. The contribution of micropores to the overall sorption process increases with the temperature increase.
Conclusion
The possibility of using oil refining waste – petroleum coke – to produce sorbent for organic compounds was studied. The activated petroleum coke with a specific surface area of 1,218 m2/g was obtained. No oxygen-containing compounds (organic acids or esters), that could be functional surface groups, were found in the activated petroleum coke.
The sorption process was described by the Langmuir, Freundlich, Temkin, and Dubinin – Radushkevich models. The sorption process is best described by the Temkin model, which not only takes into account the heterogeneity of the sorbent surface, but also the adsorbate-adsorbate interaction, which takes place in the sorbent micropores.
The sorption of ethanol on activated petroleum coke is a physical process and proceeds mainly in the sorbent micropores. As the temperature increases, the contribution of micropores to the overall sorption process increases.
The maximum sorption capacity of ethanol on activated petroleum coke was 11-15 mol/kg, which is higher than the values reported in the literature [27, 42] for activated carbons (3-5 mol/kg). The higher sorption capacity of the obtained material may be due to the higher specific surface area of activated coke compared to activated carbons. Thus, it is more economically reasonable to use the obtained material for water purification from ethanol.
The obtained value of enthalpy for the temperature range 285-300 K is –26.07 kJ/mol. The negative value of enthalpy indicates that the sorption process is exothermic. The Gibbs energy is –13.18, –12.74 and –12.24 kJ/mol for temperatures of 285, 293 and 300 K respectively. Such low values of Gibbs energy indicate that the process runs near the equilibrium.
References
- Zhichao Li, Liyuan Wu, Huijuan Liu et al. Improvement of aqueous mercury adsorption on activated coke by thiol-functionalization. Chemical Engineering Journal. 2013. Vol. 228, p. 925-934. DOI: 10.1016/j.cej.2013.05.063
- Zubkova O.S., Pyagay I.N., Pankratieva K.A., Toropchina M.A. Development of composition and study of sorbent properties based on saponite. Journal of Mining Institute. 2023. Vol. 259, p. 21-29. DOI: 10.31897/PMI.2023.1
- Fatma Oguz Erdogan, Turkan Kopac. Highly Effective Activated Carbons from Turkish-Kozlu Bituminous Coal by Physical and KOH Activation and Sorption Studies with Organic Vapors. International Journal of Chemical Reactor Engineering. 2019. Vol. 17. Iss 5. N 20180071. DOI: 10.1515/ijcre-2018-0071
- Song Ding, Yuran Li, Tingyu Zhu, Yangyang Guo. Regeneration performance and carbon consumption of semi-coke and activated coke for SO2 and NO removal. Journal of Environmental Sciences. 2015. Vol. 34, p. 37-43. DOI: 10.1016/j.jes.2015.02.004
- Kun Tong, Aiguo Lin, Guodong Ji et al. The effects of adsorbing organic pollutants from super heavy oil wastewater by lignite activated coke. Journal of Hazardous Materials. 2016. Vol. 308, p. 113-119. DOI: 10.1016/j.jhazmat.2016.01.014
- Gogolinskiy K., Vinogradova A., Kopylova T. et al. Study of physicochemical properties of polyethylene gas pipelines material with a prolonged service life. International Journal of Pressure Vessels and Piping. 2022. Vol. 200. N 104825. DOI: 10.1016/j.ijpvp.2022.104825
- Pashkevich M.А., Korotaeva A.E., Matveeva V.A. Experimental simulation of a system of swamp biogeocenoses to improve the efficiency of quarry water treatment. Journal of Mining Institute. 2023. Vol. 263, p. 785-794.
- Vasilyeva M.A., Volchikhina A.A., Morozov M.D. Re-backfill technology and equipment. Mining Informational and Analytical Bulletin. 2021. N 6, p. 133-144 (in Russian). DOI: 10.25018/0236_1493_2021_6_0_133
- Vinogradova A., Gogolinskii K., Umanskii A. et al. Method of the Mechanical Properties Evaluation of Polyethylene Gas Pipelines with Portable Hardness Testers. Inventions. 2022. Vol. 7. Iss. 4. N 125. DOI: 10.3390/inventions7040125
- Virla L.D., Montes V., Wu J. et al. Synthesis of porous carbon from petroleum coke using steam, potassium and sodium: Combining treatments to create mesoporosity. Microporous and Mesoporous Materials. 2016. Vol. 234, p. 239-247. DOI: 10.1016/j.micromeso.2016.07.022
- Rambabu N., Azargohar R., Dalai A.K., Adjaye J. Evaluation and comparison of enrichment efficiency of physical/chemical activations and functionalized activated carbons derived from fluid petroleum coke for environmental applications. Fuel Processing Technology. 2013. Vol. 106, p. 501-510. DOI: 10.1016/j.fuproc.2012.09.019
- Simin Liu, Yeru Liang, Wan Zhou et al. Large-scale synthesis of porous carbon via one-step CuCl2 activation of rape pollen for high-performance supercapacitors. Journal of Materials Chemistry A. 2018. Vol. 6. Iss. 25, p. 12046-12055. DOI: 10.1039/c8ta02838a
- Gurten I.I., Ozmak M., Yagmur E., Aktas Z. Preparation and characterisation of activated carbon from waste tea using K2CO3. Biomass Bioenergy. 2012. Vol. 37, p. 73-81. DOI: 10.1016/j.biombioe.2011.12.030
- Shida Liu, Haiyan Wang, Patrick Neumann et al. Esterification over Acid-Treated Mesoporous Carbon Derived from Petroleum Coke. ACS Omega. 2019. Vol. 4. Iss. 3, p. 6050-6058. DOI: 10.1021/acsomega.8b03472
- Jalilov A.S., Ruan G., Hwang C.C. et al. Asphalt-Derived High Surface Area Activated Porous Carbons for Carbon Dioxide Capture. ACS Applied Materials & Interfaces. 2015. Vol. 7. Iss. 2, p. 1376-1382. DOI: 10.1021/am508858x
- Wu J., Montes V., Virla L.D., Hill J.M. Impacts of amount of chemical agent and addition of steam for activation of petroleum coke with KOH or NaOH. Fuel Processing Technology. 2018. Vol. 181, p. 53-60. DOI: 10.1016/j.fuproc.2018.09.018
- Montes V., Xiao Y., Wu J., Hill J.M. Distribution of potassium during chemical activation of petroleum coke: Electron microscopy evidence and links to phase behaviour. The Canadian Journal of Chemical Engineering. 2023. Vol. 101. Iss. 5, p. 2621-2632. DOI: 10.1002/cjce.24677
- Fatma Oguz Erdogan. Characterization of the Activated Carbon Surface of Cherry Stones Prepared by Sodium and Potassium Hydroxide. Analytical Letters. 2016. Vol. 49. Iss. 7, p. 1079-1090. DOI: 10.1080/00032719.2015.1065879
- Pearson R.G. Hard and Soft Acids and Bases. Journal of the American Chemical Society. 1963. Vol. 85. N 22, p. 3533-3539. DOI: 10.1021/ja00905a001
- Pearson R.G. Absolute Electronegativity and Hardness: Application to Inorganic Chemistry. Inorganic Chemistry. 1988. Vol. 27, p. 734-740. DOI: 10.1021/ic00277a030
- Chunlan Lu, Shaoping Xu, Changhou Liu. The role of K2CO3 during the chemical activation of petroleum coke with KOH. Journal of Analytical and Applied Pyrolysis. 2010. Vol. 87. Iss. 2, p. 282-287. DOI: 10.1016/j.jaap.2010.02.001
- Hussaro K. Preparation of activated carbon from palm oil shell by chemical activation with Na2CО3 and ZnCl2 as imprenated agents for H2S adsorption. American Journal of Environmental Sciences. 2014. Vol. 10. N 4, p. 336-346. DOI: 10.3844/ajessp.2014.336.346
- Maulina S., Handika G., Irvan, Iswanto A.H. Quality Comparison of Activated Carbon Produced From Oil Palm Fronds by Chemical Activation Using Sodium Carbonate versus Sodium Chloride. Journal of the Korean Wood Science and Technology. 2020. Vol. 48. N 4, p. 503-512. DOI: 10.5658/WOOD.2020.48.4.503
- Xupei Zhu, Yi Fu, Gengshen Hu, Yang Shen et al. CO2 Capture with Activated Carbons Prepared by Petroleum Coke and KOH at Low Pressure. Water, Air, & Soil Pollution. 2013. Vol. 224. Iss. 1. N 1387. DOI: 10.1007/s11270-012-1387-y
- Eunji Jang, Seung Wan Choi, Seok-Min Hong et al. Development of a cost-effective CO2 adsorbent from petroleum coke via KOH activation. Applied Surface Science. 2018. Vol. 429, p. 62-71. DOI: 10.1016/j.apsusc.2017.08.075
- Yu Shi, Jinwen Chen, Jian Chen et al. Preparation and evaluation of hydrotreating catalysts based on activated carbon derived from oil sand petroleum coke. Applied Catalysis A: General. 2012. Vol. 441-442, p. 99-107. DOI: 10.1016/j.apcata.2012.07.014
- Delgado J.A., Águeda V.I., Uguina M.A. et al. Separation of ethanol-water liquid mixtures by adsorption on BPL activated carbon with air regeneration. Separation and Purification Technology. 2015. Vol. 149, p. 370-380. DOI: 10.1016/j.seppur.2015.06.011
- Baran P., Jodłowski G., Krzyżanowski A., Zarębska K. Experimental testing of methanol sorption on selected coal samples from Upper Silesian Basin. Geology, Geophysics & Environment. 2014. Vol. 40. N 3, p. 261-269. DOI: 10.7494/geol.2014.40.3.261
- Sergeev V.V., Cheremisina O.V., Fedorov A.T. et al. Interaction Features of Sodium Oleate and Oxyethylated Phosphoric Acid Esters with the Apatite Surface. ACS Omega. 2022. Vol. 7. Iss. 3, p. 3016-3023. DOI: 10.1021/acsomega.1c06047
- Kurdiumov V.R., Timofeev K.L., Maltsev G.I., Lebed A.B. Sorption of nickel (II) and manganese (II) ions from aqueous solutions. Journal of Mining Institute. 2020. Vol. 242, p. 209-217. DOI: 10.31897/PMI.2020.2.209
- Madero-Castro R.M., Vicent-Luna J.M., Peng X., Calero S. Adsorption of Linear Alcohols in Amorphous Activated Carbons: Implications for Energy Storage Applications. ACS Sustainable Chemistry & Engineering. 2022. Vol. 10. Iss. 20, p. 6509-6520. DOI: 10.1021/acssuschemeng.1c06315
- Gendler S.G., Fazylov I.R., Abashin A.N. The results of experimental studies of the thermal regime of oil mines in the thermal method of oil production. Mining Informational and Analytical Bulletin. 2022. N 6-1, p. 248-262. DOI: 10.25018/0236_1493_2022_61_0_248
- Hu Q., Zhang Z. Application of Dubinin-Radushkevich isotherm model at the solid/solution interface: A theoretical analysis. Journal of Molecular Liquids. 2019. Vol. 277, p. 646-648. DOI: 10.1016/j.molliq.2019.01.005
- Cheremisina O.V., Schenk J., Cheremisina E.A., Ponomareva M.A. Thermodynamic Model of Ion-Exchange Process as Exemplified by Cerium Sorption from Multisalt Solutions. Journal of Mining Institute. 2019. Vol. 237, p. 307-316. DOI: 10.31897/PMI.2019.3.307
- Cheremisina O.V., Ponomareva M.A., Bolotov V.A. et al. Thermodynamic Characteristics of the Hydrogen Sulfide Sorption Process by Ferromanganese Materials. ACS Omega. 2022. Vol. 7. Iss. 3, p. 3007-3015. DOI: 10.1021/acsomega.1c06037
- Gendler S.G., Fazylov I.R. Methods of regulation air temperature in the Russian oil mains. Topical Issues of Rational Use of Natural Resources, 2019. Vol. 1. London: CRC Press, p. 16-21. DOI: 10.1201/9781003014577
- Tagirov M.A., Zhirnov B.S., Gostkov E.V. et al. Activation of petroleum coke to obtain catalyst substrates. Coke and Chemistry. 2011. Vol. 54. Iss. 10, p. 379-382. DOI: 10.3103/S1068364X11100103
- Ronglin Xiao, Shaoping Xu, Qingxue Li, Yanmin Su. The effects of hydrogen on KOH activation of petroleum coke. Journal of Analytical and Applied Pyrolysis. 2012. Vol. 96, p. 120-125. DOI: 10.1016/j.jaap.2012.03.013
- Lee S.H., Choi C.S. Chemical activation of high sulfur petroleum cokes by alkali metal compounds. Fuel Processing Technology. 2000. Vol. 64. Iss. 1-3, p. 141-153. DOI: 10.1016/S0378-3820(00)00070-9
- Mingbo Wu, Qingfang Zha, Jieshan Qiu et al. Preparation of porous carbons from petroleum coke by different activation methods. Fuel. 2005. Vol. 84. Iss. 14-15. p. 1992-1997. DOI: 10.1016/j.fuel.2005.03.008
- Kudinova A.A., Poltoratckaya M.E., Gabdulkhakov R.R. et al. Parameters influence establishment of the petroleum coke genesis on the structure and properties of a highly porous carbon material obtained by activation of KOH. Journal of Porous Materials. 2022. Vol. 29. Iss. 5, p. 1599-1616. DOI: 10.1007/s10934-022-01287-1
- Hajilari M., Shariati A., Khosravi-Nikou M. Mass transfer determination of ethanol adsorption on activated carbon: kinetic adsorption modeling. Heat and Mass Transfer. 2019. Vol. 55. Iss. 8, p. 2165-2171. DOI: 10.1007/s00231-019-02575-4