On the possibility of reducing man-made burden on benthic biotic communities when mining solid minerals using technical means of various designs
Abstract
The paper analyses features of the species composition and diversity of biotic communities living within the ferromanganese nodule fields (the Clarion-Clipperton field), cobalt-manganese crusts (the Magellan Seamounts) and deep-sea polymetallic sulphides (the Ashadze-1, Ashadze-2, Logatchev and Krasnov fields) in the Russian exploration areas of the Pacific and Atlantic Oceans. Prospects of mining solid minerals of the world’s oceans with the least possible damage to the marine ecosystems are considered that cover formation of the sediment plumes and roiling of significant volumes of water as a result of collecting the minerals as well as conservation of the hydrothermal fauna and microbiota, including in the impact zone of high temperature hydrothermal vents. Different concepts and layout options for deep-water mining complexes (the Indian and Japanese concepts as well as those of the Nautilus Minerals and Saint Petersburg Mining University) are examined with respect to their operational efficiency. The main types of mechanisms that are part of the complexes are identified and assessed based on the defined priorities that include the ecological aspect, i.e. the impact on the seabed environment; manufacturing and operating costs; and specific energy consumption, i.e. the technical and economic indicators. The presented morphological analysis gave grounds to justify the layout of a deep-sea minerals collecting unit, i.e. a device with suction chambers and a grip arm walking gear, selected based on the environmental key priority. Pilot experimental studies of physical and mechanical properties of cobalt-manganese crust samples were performed through application of bilateral axial force using spherical balls (indenters) and producing a rock strength passport to assess further results of the experimental studies. Experimental destructive tests of the cobalt-manganese crust by impact and cutting were carried out to determine the impact load and axial cutting force required for implementation of the collecting system that uses a clamshell-type effector with a built-in impactor.
Introduction
Ores that form accumulations on the ocean floor are not only of significant scientific interest [1-3], but also of practical significance associated with studying the possibility of their recovery [4-6]. Work under contracts between the Russian Federation and the UN International Seabed Authority is in progress for the following three types of ocean solid commercial minerals (SCM) in the international seabed areas, i.e. ferromanganese nodules (FMN) in the Russian Exploration Area (REA-FMN) within the Clarion-Clipperton Field (Pacific Ocean); cobalt-manganese crust (CMC) in the Russian Exploration Area (REA-CMC) within the Magellan Seamounts (Pacific Ocean) and deep-water polymetallic sulphides (DPS) in the Russian Exploration Area (REA-DPS) within the Mid-Atlantic Ridge (MAR). The main challenges in addressing the possible development of ocean mineral resources include the following: designing of deep ocean mining equipment (collecting units); protection and preservation of the marine environment, including the unique ecosystems comprising the diverse biological communities of ore fields in the abyssal plains, mid-ocean ridges and seamounts of the world's oceans [7].
Problem statement
The entire scope of specific features related to the species composition and diversity of benthic life communities in the world's oceans have to be investigated in order to justify the rational parameters of technical means, which will minimize the impact on the benthic ecosystem during the commercial mining of deep-sea solid minerals, i.e. the ferromanganese nodules, cobalt-manganese crust and deep-water polymetallic sulphides. The description of benthic organisms was based on the archive materials of the VNIIOkeangeologia Research Institute obtained during the JSC Polar Marine Exploration Expedition (PMGRE) and the JSC “Yuzhmorgeologia” voyages.
Subsea fauna of the ferromanganese nodule areas
The ferromanganese nodules is a new type of mineral raw materials that contains strategically important metals for the ferrous (Mn) and non-ferrous (Ni, Cu, Co) industries. These nodules have the same mineral content and the resource potential as the currently mined surface ore fields, while having the advantage of their complex composition and sorption properties [8].
Ferromanganese nodules in the Russian Exploration Area are confined to the Clarion-Clipperton ore province with the size of about 5000 ´ 10000 km located in the central abyssal basin of the Pacific Ocean. Over a half of the province is a flat horizontal field at the depths of 4000-6000 m, which terrain is complicated by individual meridionally oriented ridges and seamounts up to 2500 m high. Sedimentary rocks below the depths of 4200-4500 m, i.e. the critical depth of carbonate accumulation, are represented exclusively by clays; above this depth, carbonate rocks can be encountered [9]. The field is inhabited by diverse representatives of epifauna, which provide habitat for other species.
Expeditions of JSC Yuzhmorgeologia and foreign publications [10-12] report representatives of macrofauna (numerous Polychaeta worms as well as Tanaidacea and Isopoda crustaceans), meiofauna (Nematoda filiform worm and Harpacticoida crustaceans), epifauna (representatives of the Stephanoscyphus Scyphozoa hydranth in the polypiform stage, colonies of bryozoans, xenophyophorids living in calcareous tubes of the Serpulidae Polychaeta worms) and infauna of the nodules (mainly shell-less foraminifera). One of such organisms is the transparent anemone (Losactis vagabunda), which makes its way through the sediments and can ingest worms six times its own weight. Relatively large animals on the abyssal plains include sea cucumbers, sea urchins, and starfish (Fig.1).
Despite the absence of large marine life communities and the favourable morphology of the ore deposits, the idea of large-scale mining of nodule deposits is actively opposed by some experts, who believe that nodule mining could lead to significant damage to habitats and to extinction of unique species. The physical recovery of manganese nodules takes millions of years [13]. The argument is based on observations made 26 years later at an experimental nodule mining site within the Clarion-Clipperton Fracture Zone. Traces of the mining equipment were still clearly visible, and communities of the benthic life had not fully recovered [14].
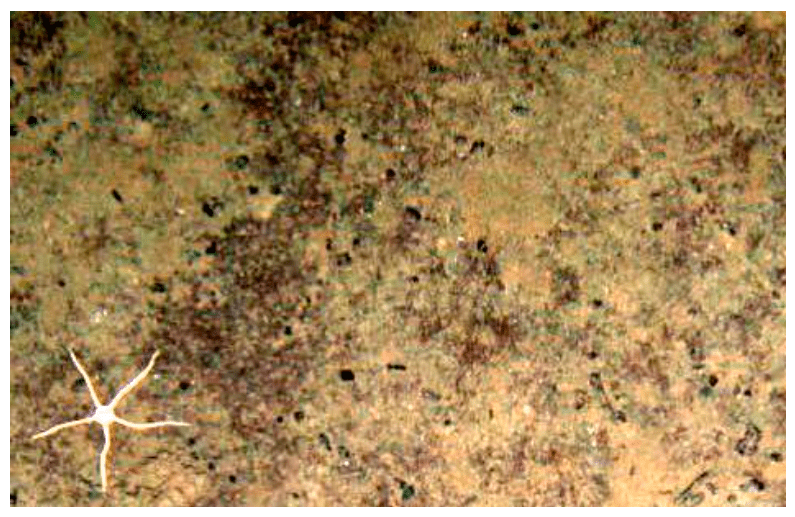
Fig.1. Ferromanganese nodules in the Clarion-Clipperton field, Pacific Ocean (13° N, 133° E)
Subsea fauna of the cobalt-manganese crust areas
Cobalt-manganese crust of the Russian Exploration Area is found on the Magellan Seamounts at the depths of 1400-3500 m [15]. Cobalt-manganese crust is formed on the slopes of large tablemounts, or guyots, rising over a kilometre above the seafloor. The apex plateau of a guyot is typically covered with loose carbonate sediments and is an ore-free zone. At the boundaries of the apex plateau, the gently sloping surfaces with gradients from 0-5° to 12-20° are almost always free of sediments, and the thickest layers of the cobalt-manganese crust are encountered on the bedrock outcrops. These are usually continuous undisturbed covers, rarely topped with carbonate sediments. Approximately 2/3 of the seabed surface is covered with solid substrate in the form of crust formations or ferromanganese nodules, sometimes with a small amount of loose sediments (Fig.2, a). The rest of the surface is mainly topped with a thin layer of sediments. The cobalt-manganese crust is found on exposed bedrock surfaces, while the nodules are confined to areas of unlithified sediments (Fig.2, b). Seamounts of the Cretaceous age, which tops are located at the maximum depth of 1400-1600 m, are considered the most promising for discovery of rich cobalt-manganese crust ore fields [16].
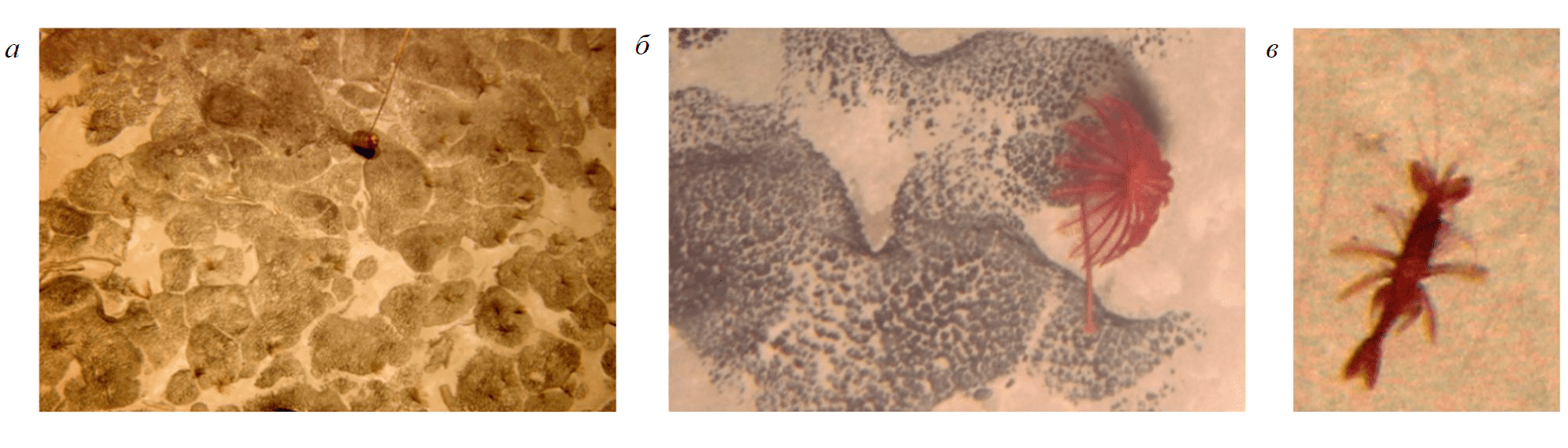
Fig.2. Comatulidae sea lilies (a) and Proisicrinus ruberrimus (b) (Guyot Alba, 16°52' N, 154°4' E); Aristaemorpha crustaceans(Aristidae) (c) (Guyot Kotzebue, 17°25' N, 153°10' E)
The composition and diversity of benthic fauna depend on the depth of the producing slopes and the amount of sediments. Seamounts at the depths of three and more kilometres are similar to the abyssal plains in terms of their ecosystems. The shallower guyots, characterized by the presence of corals, anemones, feather stars and sponges, are sometimes compared to oases, which are used by some of the migratory species as feeding areas [17]. It is therefore recommended to classify seamounts as sensitive marine ecosystems [18].
It should be noted that the benthic fauna of the guyots is almost exclusively concentrated on their flat tops covered with sediments, which are the primary areas of industrial interest.
Frequent predominance of sea lilies is noted in the expeditions of JSC “Yuzhmorgeologia” and in foreign publications [7, 18]. The Decapoda crustaceans are observed among the animals (Fig.2, c). Large-size decapods are presented by the Nematocarcinidae and Polychelidae families. The sea-pen coral polyps (Pennatulacea) are relatively abundant in the seabed areas covered with sediments. There can also be found sea anemones (Actiniaria), tube anemones (Ceriantharia), soft corals (Alcyonacea), glass sponges (Hexactinellida) and ray-finned fish (Actinopterigii).
The numbers of large-size representatives of the surface fauna are quite low (not exceeding 70-100 individuals per hectare). Smaller-size specimens of the deep-sea benthos that inhabit the sediments are more abundant, particularly the meiofauna organisms, which number can reach up to 3000 individuals per 0.25 m2. However, the thickness of sediments on top of the guyots rarely exceeds 10 cm.
Subsea fauna of the deep-sea polymetallic sulphides areas
Deposits of the deep-water polymetallic sulphides are widespread and are associated with different geological and geomorphological conditions. The considered hydrothermal fields are related to the crust spreading centres or mid-ocean ridges. The hydrothermal systems within such structures can be attributed to both ultrabasic rocks of Internal Oceanic Complexes (IOC) and basalts. The fields can be located within a wide range of depths (from the first hundred to 4000 m and deeper) within various geomorphological structures, i.e. in the central axial part of a mid-ocean ridge; on the flank of a mid-ocean ridge; on the slope of a rift valley; within the transform faults and intraplate hotspots.
The presence of active hydrothermal processes is a major factor determining the diversity of species found near the deep-sea hydrothermal systems in the world's oceans. The hydrothermal solutions circulating through the fracture system are enriched with numerous elements as the result of serpentinization, leaching, and other processes. High concentrations of such elements as H2S, H2, CH4, NH3, Mn2+ and Fe2+ support microbial metabolism in the hydrothermal systems and plumes [19-21]. The hydrothermal plumes are the result of interaction between the “nutrient” hydrothermal fluid and the surrounding seawater [22].
The hydrothermal biotic communities found within the Mid-Atlantic Ridge are no exception. They are usually dominated by the Bathymodiolus spp. bivalve mollusk, Rimicaris exoculata blind shrimp, which form large-size colonies on the walls of the “black smokers”, various Polynoid polychaete worms, Segonzacia mesatlantica crabs and some numerically insignificant hydrothermal fish species. Close relationships between a primary bacterial producer and a consumer explain much of the biological abundance of the deep-sea hydrothermal vents.
Recent results of modelling the distribution of active hydrothermal systems show that the number of undiscovered hydrothermal fields is highly underestimated (at least by a factor of 3-6). The re-assessment would revise the distribution pattern of deep-sea hydrothermal fauna and increase the biogeochemical contribution of hydrothermal sources (including the low-temperature diffusive ones) [23, 24]. Studies have shown that even inactive hydrothermal systems with extensive deep-water polymetallic sulphide deposits may be of interest to the mining companies. Such areas are also characterized with endemic associations of organisms that need to be protected and preserved during mining of the deep-water polymetallic sulphides [25].
Four typical hydrothermal sites can be identified within the deposits of deep-water polymetallic sulphides (DPS) in the Russian Exploration Area, which are differentiated by their hydrothermal activity, the number and density of hydrothermal fauna, as well as the species diversity of the communities studied.
1. Ashadze ore cluster, Ashadze-1 ore field
Active hydrothermal activity in the Ashadze-1 field was studied in detail from the ROV Victor 6000 remote controlled multifunctional vehicle during the SERPENTINE Russian-French expedition on board the “Pourquoi Pas?” research vessel in 2007 [26-28].
The Ashadze-1 hydrothermal field on the Mid-Atlantic Ridge is the deepest one (4080 m), and is located within the stepped-and-faulted ultramafic rocks that make up the inner oceanic complexes, i.e. serpentinites, harzburgites (peridotites), and pyroxenites. The hydrothermal activity is controlled by deep tectonic faults (detachments) [29].
Low-temperature vents (up to 113 °C) with low flow rates are observed in most parts of the field. They are accompanied by a large number of inactive sulphide pipes covered with yellow oxides; there are black coloured structures characteristic of the fresh sulphides of slightly active “smokers” (Fig.3, a). However, most of the hydrothermal structures are confined to a few vents with the temperatures above 347 °C. The increased acidity of hydrothermal solutions (pH = 2-4) should be noted.
In terms of the species composition and diversity, the Ashadze-1 hydrothermal field displays some interesting biological features. Numerous populations of symbiotic species, in particular, Rimicaris exoculata, are almost completely absent there [26]. At the same time, two species, which in other areas are known as the peripheral species, form large populations that colonize the available environment, i.e. anemone (actinia, mainly Maractis rimicarivora) and chaetopteridae (Fig.3, a).
The following three shrimp species are present on the outer walls of the high-temperature “smokers” in descending order in terms of their abundance: Mirocaris fortunata, Chorocaris chacei and Rimicaris exoculata. The latter species is usually found on the Mid-Atlantic Ridge in clusters of several thousand organisms, but only seven individuals were found [26].
Small-size populations of Ashinkailepas (aff) briandi gastropods (about 10 organisms), flatworms, and bacterial mats were found in the oxidized and active zones of the diffusion low-temperature discharge. The temperature was about 10 °C. In the oxidized zones, a large number of chaetopteridae tubes, probably Spiochaetopterus, were observed. These tubes make up large clusters at the base of the “smokers” and are used as a substrate for other species and the bacterial mats. A variety of associated fauna was also observed [26].
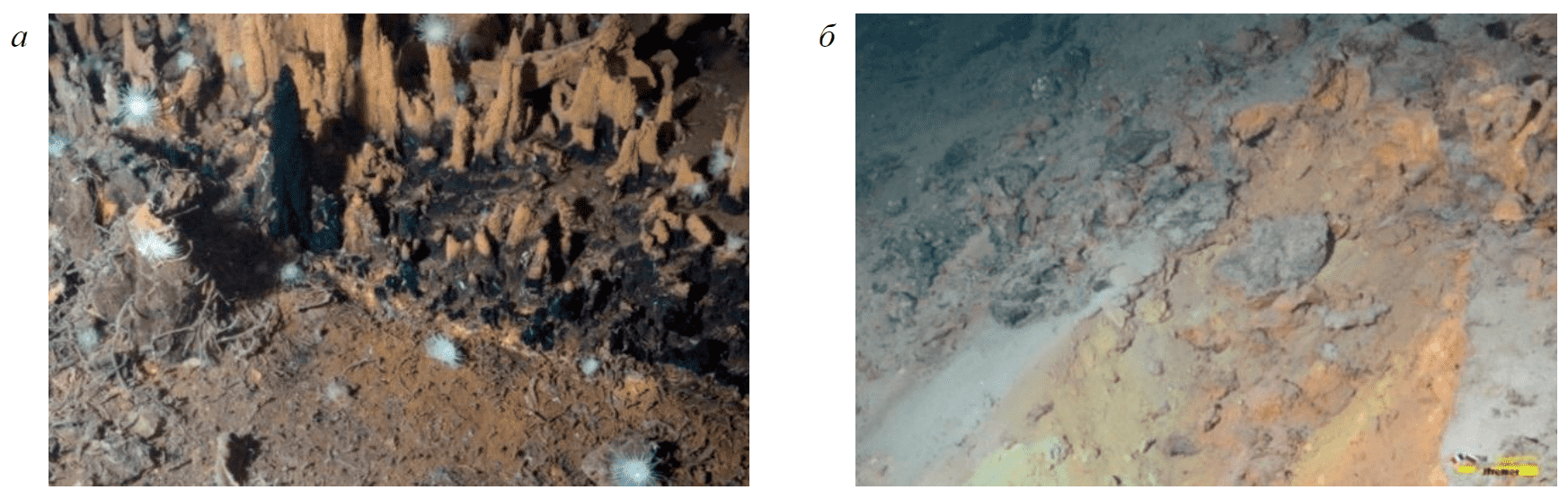
Fig.3. The seabed topography at the Ashadze-1 site, dominated by disintegrated material of decaying inactive smoker tubes and a population of the Maractis rimicarivora sea anemones and the Chaetopterids worm tubes (12°58' N, 45°30' W) (a); oxidized sulphide ores of the Ashadze-2 field (12°58' N, 45°30' W) (b) [26]
2. Ashadze ore cluster, Ashadze-2 hydrothermal field
The Ashadze-2 field is a poorly studied hydrothermal crater and an adjacent ridge of slightly sloping hills on the western side of a rift valley of the Mid-Atlantic Ridge within the same internal oceanic complex as the Ashadze-1 field. The hills are composed of sulphides of various oxidation levels (Fig.3, b). However, hydrophysical sounding showed the presence of hydrophysical anomalies within this field. The host rocks and tectonic conditions are similar to the previously described Ashadze-1 field [29].
In the central part of the hydrothermal crater there is a relatively inaccessible area of high-temperature discharge, where only individual Mirocaris fortunata shrimps were found. In the fracture zone around the crater with “black smokers” there is a diffusion zone with unusual composition of life communities and the temperature above 12 °C.
Various sponges and a population of mollusks were observed among the rare representatives of fauna. The stone blocks were covered with an unusual jelly coat of unknown origin. This atypical formation had a limited distribution and is probably related to organic enrichment [26].
3. Logatchev hydrothermal field
The Logatchev field was discovered in 1993-1994, during the seventh voyage of the “Professor Logatchev” research vessel [30]. The field was repeatedly investigated by international expeditions, including those using manned subsea vessels [29]. The most complete studies of the field that included bottom water sounding, detailed mapping, photo and video recording, geological and biological sampling were conducted from the Alvin crewed deep-ocean research submersible during the DIVERSEXPEDITION expedition onboard the Atlantis research vessel (2001) and from ROV Victor 6000 during the Russian-French SERPENTINE expedition onboard the “Pourquoi Pas?” research vessel in 2007 [26, 27, 31].
Seven groups of “black smokers” and hydrothermal craters with the solution temperatures of 348-352 °С as well as a large number of diffusion seepage zones of warm water with the temperatures of 10-20 °C are distributed over the entire area of the field. All the discharge zones are colonized by numerous biological communities [32]. The geological and tectonic conditions are similar to those of the Ashadze hydrothermal field. The Logatchev hydrothermal field is also confined to gabbro-peridotite rocks of the internal oceanic complex, and its hydrothermal activity is controlled by the detachments [33].
The most remarkable characteristics of this hydrothermal field include a large number of Bathymodiolus puteoserpentis mussels, Phymorhynchus ovatus gastropods, and clusters of Rimicaris exoculata blind shrimps (Fig.4, a). The Chorocaris chacei and Alvinocaris sp. shrimps are less abundant. Another typical feature of the Logatchev field areas is the high abundance of Ophioctenella acies brittle stars. Studies have shown a growth in populations of different species, e.g. the mussel biomass has increased, including that in the zones of diffusive discharge of the “twinkling” warm solutions.
This field includes one of the largest ore bodies of the deep-water polymetallic sulphides within the Russian Exploration Area, composed mainly of massive oxidized iron sulphides (Fig.4, b). Detailed bathymetric survey and sampling of the Krasnov field was first performed during the Russian-French SERPENTINE expedition onboard the “Pourquoi Pas?” research vessel in 2007 [26]. The field is confined to basalts and is located at the interface of the eastern side of a rift valley and a nontransform discontinuity at the depths of 3700-4000 m [33].
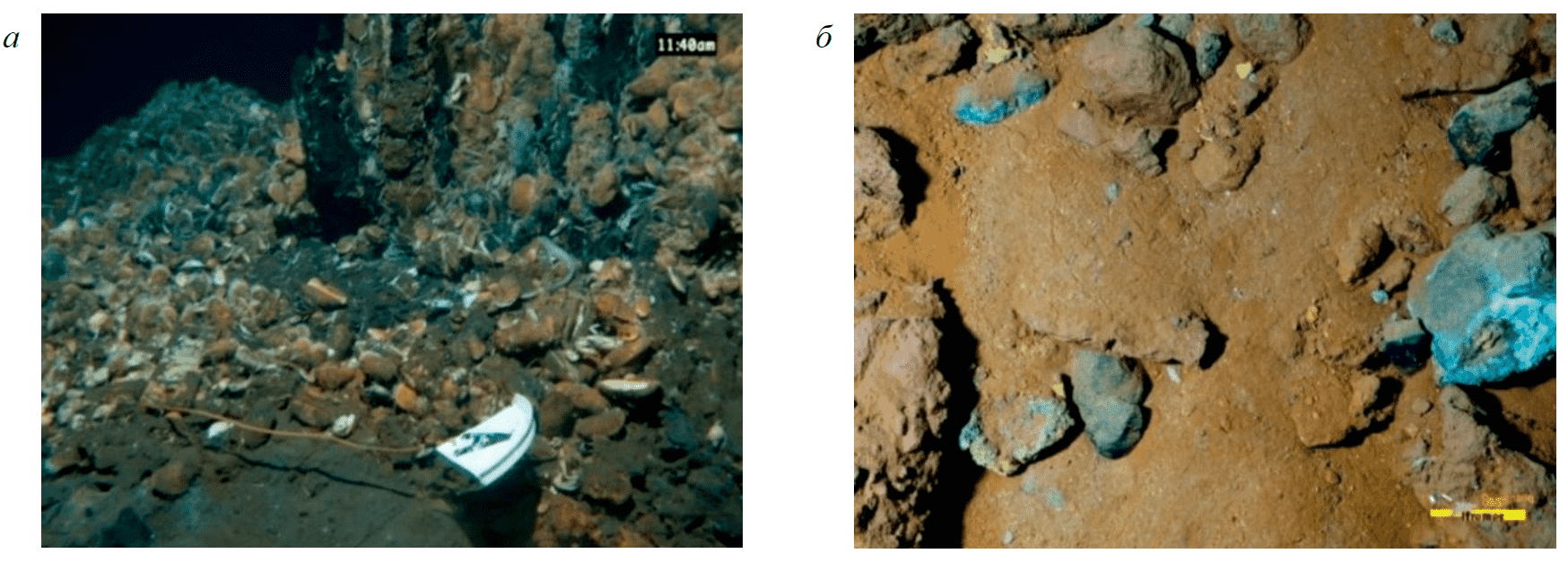
Fig.4. Logatchev field: shrimps, crabs, mussels, and gastropods near the Irina-2 vent group (14°45,17' N, 44°58,75' W), DIVERSEXPEDITION Expedition (a) [31]; Krasnov field, SERPENTINE Expedition (b) [26]
Hydrothermal activity was not observed in the Krasnov field, so the fauna usually associated with hydrothermal discharge was not detected. Nevertheless, rather thick layers of bacterial mats were registered. These observations suggest the possibility of warm solution discharging through the loose strata of oxidized sulphides. In addition, an anemone, whose morphology resembles Boloceroides daphnae described in the hydrothermal fields of the East Pacific Rise, was found in one of the cavities within the sulphide-oxide strata (the first observation of this anemone in the Mid-Atlantic Ridge).
The given typification of the hydrothermal fields based on the distribution features of benthic organisms, the nature of ore clusters and the hydrothermal activity allows us to make conclusions regarding the possibility of mining these mineral resources.
- High temperature and aggressive acidic (pH = 2-4) fluids of the “black smokers” in the areas of the increased hydrothermal activity (such as the Logatchev field) make operation of machinery for mining of the deep-water polymetallic sulphides more challenging. For this reason, creation of equipment that would be resistant to the high-temperature aggressive solutions to operate in these conditions is currently not possible.
- The abundant and unique fauna of active hydrothermal fields does not allow the use of available mechanisms for mining operations in the zone of active “smokers” in the fields of high and medium activity (such as the Logatchev and the Ashadze-1 fields). Ore mining in these conditions is impossible without inflicting serious damage to the colonies of hydrothermal communities of living organisms close to the deep-water hydrothermal systems of the ocean. At the same time, it is possible to select inactive areas available for mining in the fields of low and medium activity (such as the Ashadze-1 and the Ashadze-2 fields).
- The absence of high-temperature discharge of ore-forming solutions and the hydrothermal fauna in inactive hydrothermal fields (such as the Krasnov and the Ashadze-2 fields) makes mining of the mineral resources most favourable using the machinery, developed also for mining of the ferromanganese nodules and the cobalt-manganese crust accumulations.
- It should be noted that a certain amount of benthic organisms, especially microbiota, is also present in inactive hydrothermal fields. Therefore, it is very important to select specific effectors that would minimize the mechanical impact on the seafloor when choosing the technology and mechanical means for mining cobalt-manganese crust, ferromanganese nodules and deep-water polymetallic sulphides.
Therefore, the geological and geomorphological occurrence conditions of the main solid minerals of the World Ocean, e.g. the ferromanganese nodules, cobalt-manganese crust, and deep-water polymetallic sulphides, are fundamentally different. Whereas the ferromanganese nodules are located in relatively levelled areas, which simplifies their mining, the cobalt-manganese crust is often found on the slopes of guyots. The deposits of deep-water polymetallic sulphides are characterized by the most challenging conditions. They typically feature a wide variety of occurrence depths, as well as the host rocks and structures to which they are confined. In addition to the contrasting differences in the occurrence conditions, the areas of prospective mining of different solid minerals fundamentally differ in the character of benthic ecosystems near the deposits. Considering all these conditions, we can make the conclusion that it is currently impossible to create a universal complex to mine all the mentioned above solid minerals of the ocean that would ensure preservation of the benthic ecosystems.
Methodology
Examination of mining equipment that would minimize the environmental impact should be done for the cobalt-manganese crust and ferromanganese nodules collecting units, since their technical implementation is possible.
The Nautilus Minerals concept has been developed and tested for industrial mining of solid minerals, which consists in the use of technical solutions developed for the onshore mining and adapted for deep-water applications [34]. The Japanese concept, which involves the use of a crawler-based sea-floor assembly with the milling-type effectors, is being actively developed [35, 36]. These concepts can have a significant impact on the environment.
Tables 1 and 2 present an analysis of design options for the deep-sea equipment intended to collect ferromanganese nodules and cobalt-manganese crust, with an impact assessment of the ten most important parameters of each specific device within the most relevant concepts. The scores (1 – maximum, 0 – minimum) are the numerical representation of the analysis performed by experts and specialists of VNIIOkeangeologia Research Institute and researchers of Saint Petersburg Mining University involved in deepwater mining. The scores show the most preferable technical solution depending on its parameters and functions performed. In order to understand how a particular function and device will meet the requirements, i.e. its environmental impact and reasonable technical and economic performance indicators, a coefficient of significance is introduced, which will not be equal.
In cases where it is required to develop and justify the movement gear (Table 1), the environmental safety will be the key priority, as it is possible to design devices that would bring the impact on the environment down to an acceptable level.
In cases where the minerals collection systems are developed and justified (Table 2), a dependence was identified that by minimizing the impact on the environment, we reduce the viability and profitability of mining these minerals. There do not exist even conceptual designs for devices that can acceptably minimize the impact on the environment. Therefore, the collection systems will be developed as a balance between the two priorities, i.e. environmental safety and technical and economic performance. Table 2 presents both of the importance factors and the overall estimates that take these factors into account and which can be used when selecting the equipment to collect deep-sea solid minerals that show high technical and economic performance and have minimal impact on the environment.
Table 1
Movement gear of the sea-floor assembly (with environmental safety as the key priority)
Function |
Importance factor |
Machines with manipulators |
Walking type machines |
Crawler-based machines |
|
Possibility to fit various collecting mechanisms |
0.3 |
1 |
0.7 |
1 |
|
High travel speed |
0.7 |
0.8 |
0.6 |
1 |
|
Machine stability during operation |
0.6 |
0.9 |
0.8 |
0.9 |
|
Simple design of key assemblies |
0.5 |
0.7 |
0.8 |
0.6 |
|
Preservation of near-bottom areas |
1 |
1 |
0.5 |
0.4 |
|
High collection performance |
0.8 |
0.7 |
0.8 |
1 |
|
Availability of analogous key assemblies in the industry |
0.4 |
0.8 |
0.7 |
1 |
|
Prevention of contamination with finely dispersed silt |
0.9 |
0.9 |
0.4 |
0.3 |
|
Overall estimate |
|
4.44 |
3.29 |
3.71 |
Table 2
Bottom minerals collection systems
Function |
Importance factor |
Machines with manipulators and grippers designed as suction chambers |
Machines with hydraulic rippers and gripping water jets |
Machines with gathering elements (scrapers, arms, chains) |
Augers, ripper bars, disks, |
Buckets, rope-driven |
|
High yield |
1**, 0.5* |
0.6 |
0.7 |
1 |
0.8 |
0.5 |
|
Possibility and simplicity |
0.2**, 0.6* |
1 |
0.8 |
0.7 |
0.7 |
0.6 |
|
Minimum mechanical impact |
0.3**, 1* |
0.9 |
0.5 |
0.6 |
0.7 |
0.5 |
|
Minimal roiling |
0.5**, 0.9* |
0.9 |
0.5 |
0.6 |
0.6 |
0.5 |
|
Low specific energy consumption |
0.8**, 0.4* |
0.8 |
0.7 |
0.9 |
0.7 |
0.6 |
|
High efficiency |
0.9**, 0.3* |
0.6 |
0.7 |
0.8 |
0.8 |
0.6 |
|
Simplicity of its own design and mounting on the delivery vessel |
0.6**, 0.2* |
0.6 |
0.7 |
0.8 |
0.8 |
0.9 |
|
Minimal manufacturing cost |
0.7**, 0.8* |
0.7 |
0.7 |
0.9 |
0.8 |
0.9 |
|
Possibility to pick up and carry the materials within the dimensions of the collecting unit |
0.3**, 0.7* |
0.9 |
0.5 |
0.9 |
0.8 |
0.9 |
|
Overall estimate |
|
3.92**/4.52* |
3.59**/3.4* |
4.52**/4.25* |
4.09**/3.7* |
3.58**/3.61* |
Note. * – meets the environmental impact top priority; ** – meets the technical and economic performance top priority.
The priority in terms of environmental impact (roiling, mechanical impact on the sea floor) puts the devices with suction chambers and grip arm walking gear on high places; in terms of technical and economic indicators (cost, yield, efficiency), concepts with the crawler gear and hydraulic transport appear to be the optimal ones.
In order to create a feasible design for collecting deep-sea ferromanganese nodules and cobalt-manganese crust, the following three options should be considered:
- for small and medium-sized ferromanganese nodules (up to 100 mm) in the absence of unique flora and fauna forms;
- for ferromanganese nodules and cobalt-manganese crust with a significant variation in particle sizes (50-250 mm) in the presence of abundant unique forms of flora and fauna;
- for uniform cobalt-manganese crust with complex occurrence conditions on guyot slopes in the presence of unique flora and fauna forms.
The concept with a crawler-based collecting unit and a vertical hydraulic transport is applicable for the first option. For example, the Indian concept that uses a crawler-based collecting unit with a drum effector, a conveyor and a flexible duct that transports the collected ferromanganese nodules to the supply vessel using a pumping unit, perfectly meets these requirements [37-39]. It is also necessary to consider the design options that use hydraulic transport with an intermediate pumping capsule [40].
The second option can use the collecting unit designed at the Saint Petersburg Mining University (Fig.5, a) with effectors that use suction chambers 1, which are able to suck in large nodules and move them into the bunker. The suction chambers (Fig.5, b) are shaped as hollow plates 2 where lower pressure (relative to the external pressure) is created, with suction cups 3 on the working face that collect the minerals 4 due to the pressure difference. The suction chambers work in cycles by pressing against the seafloor, sucking in the nodules and then pulling away from the seafloor, carrying the collected material into the bunker.
The third option can also use the concept of developed at the Saint Petersburg Mining University. The difference consists in replacing the suction chambers fixed to arms 5 with clamshell-type buckets 6 equipped with impactors (Fig.5, c) [41]. The efficiency of the clamshell-type effector (Fig.5, d) with a minimum impact on the environment will be achieved by selective and controlled collection without damage to the substrate. In case the cobalt-manganese crust has significant hardness, and the axial pressing force is not sufficient to introduce and clamp jaws 8, it is proposed to preliminarily break up the massif with a built-in impactor 7, which will allow separating the cobalt-manganese crust from the substrate without additional dilution.
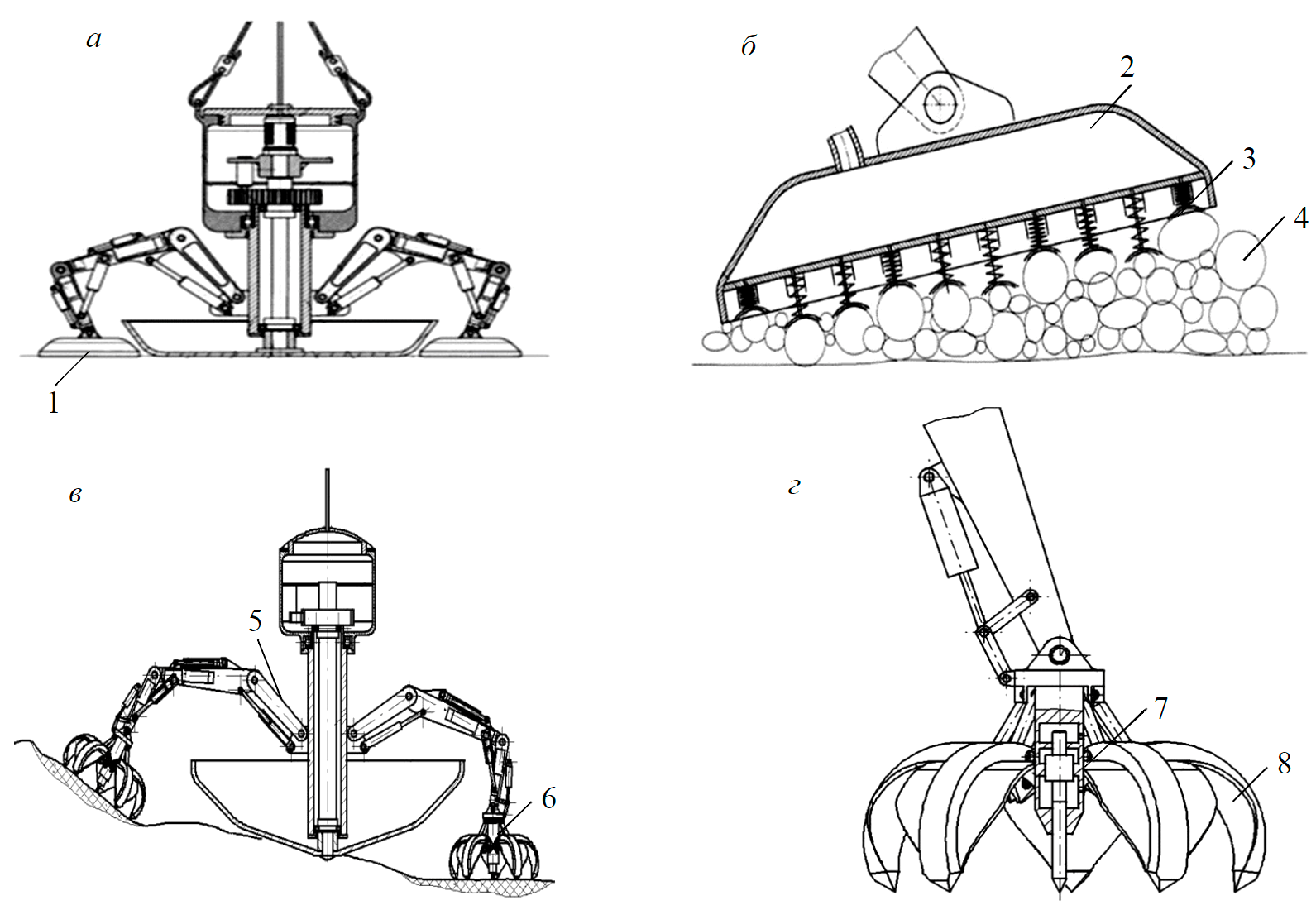
Fig. 5. A walking-type collecting unit with suction chambers as effectors (a) and the design of a suction chamber in close view [42, 43] (b); a walking-type collecting unit with a clamshell-type effector (c) and the design of the clamshell effector with a built-in impactor [44] (d)
A design concept of the deep-water complex with the collecting unit proposed by the Saint Petersburg Mining University that provides improved environmental performance is shown in Fig.6, a.
The mining system includes: an supply vessel 8 with two winches located fore and aft; self-propelled platforms 3 to accommodate the stationary equipment; power supply equipment including a transformer 6, a hydraulic powerpack 5 and a winch 4; a self-propelled collecting unit 13; a self-propelled feeder 12; a bucket (or a bunker) 11.
The connection of the collecting unit with the power supply equipment is accomplished through the winch 4, which carries an umbilical cable 2 to supply the collecting unit with electrohydroenergy. The power supply equipment is connected to the vessel by means of a high-voltage self-supporting cable 7. Lowering and lifting of the bucket is carried out by a gripping mechanism 10. The gripping mechanism has maneuvering devices 9 that ensure its accurate positioning during lowering and lifting.
The recovered minerals are transferred from the bucket to the vessel's storage bin, the material being pre-processed by separating the liquid part and dewatering. Further transportation can be done using an additional barge, on which the dried minerals are reloaded, or by the supply vessel itself 8.
Positioning and orientation of all the units and devices takes place with the help of transceivers 1, spaced at a certain pitch and forming a coordinate grid. All signals from the transceivers are routed to the central control unit located onboard the supply vessel, which sends the response control signals to the transceivers, and they forward the signals to the units and devices involved in the collection of minerals.
Other transport systems can be used, e.g. those using a screw feeder 14 and a closed-type bucket 15 connected to the duct of the hydraulic transport system (Fig.6, b); lifting by means of a propeller-driven bucket 17 attached to a cable 16 (Fig.6, c); using a skip hoisting system in which the skips 19 are loaded with a chain conveyor 20, lifted to the supply vessel and unloaded in the hoist head frame 18 (Fig.6, d). Application of the latter system is possible when the waters are calm or with the use of special rolling and pitching compensation systems.
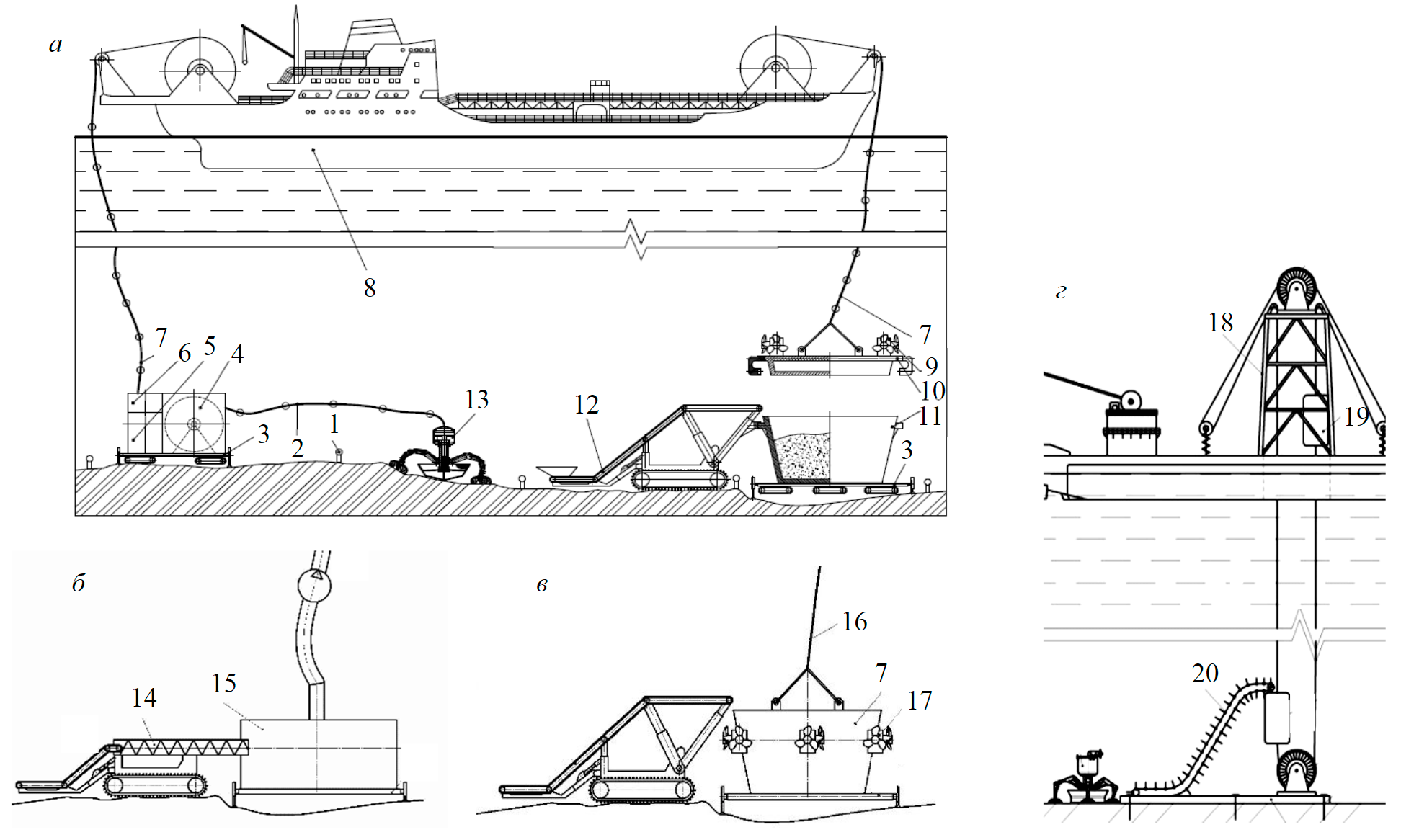
Fig.6. A concept for cobalt-manganese crust and ferromanganese nodule mining system with a walking-type collecting unit proposed by Saint Petersburg Mining University (a); options for the hydraulic transport system (b), bucket (c) and skip hoisting (d)
When assessing the output of the complex, the key factor is the parameters and the type of collecting unit, which will control the mining performance.
The estimated per hour output of one collecting unit should be calculated using the following equation [41]
where ρ is the density of the mineral; E is the intermediate bunker volume; Tcol is the collecting time per effector; Tload is the time to load one bucket into the bunker; n is the number of effectors required to fill the bunker; Ttrav is the travel time to the reloading station; Tunload is the time to unload the bunker onto the reloader; Treturn is the travel time to the previous or new collection point.
Finding the Tload, Ttrav, Tunload and Treturn values consists in calculating the parameters related to the operating time of the hydraulic cylinders of the effectors. Each operation can be calculated as a combination of hydraulic cylinders working simultaneously or sequentially only once the required hydraulic cylinder type has been selected for each particular operation. The order and degree of extension of the hydraulic cylinder rods is calculated using the designed planogram of the collecting unit. In order to determine the timing of Tcoll, it is necessary to know the physical and mechanical properties of the minerals and the forces at the effector. The choice of effector dimensions will also depend on the occurrence characteristics of the deposits (their average thickness).
The number of collection operations n required to fill the bunker depends on the volume of the bunker and the number of the collecting units. This problem is basically solved at the feasibility study phase of the field mining system, which should maximize the uninterrupted operation of the transport system.
Based on the geological data, to ensure profitability it is required to keep the production rate of 1 million t/year of minerals, which corresponds to 150-250 t/h.
Discussion
In order to verify the possibility of using a clamshell-type effector to collect the cobalt-manganese crust with a minimum environmental impact, experimental tests were performed to determine the basic regularities in cobalt-manganese crust breaking by cutting, i.e. through introduction of the clamshell bucket, and by impact pulses, i.e. separation of the cobalt-manganese crust from the substrate. Since no definite data on physical and mechanical properties of the cobalt-manganese crusts are available, and their structure and parameters may vary with the occurrence depth, it is necessary to specify physical and mechanical properties of the available samples before conducting the experimental tests with rock-cutting tools on the designed test benches. For this purpose a calculation method was used that was based on the parameters experimentally obtained by loading the samples with spherical indenters [45], and building the strength data sheet of the cobalt-manganese crust in three states: air-dry, as is (20 % moisture content), and water-saturated (Table 3).
Table 3
Definition of physical and mechanical properties of cobalt-manganese crust samples with spherical indenters
Defined property |
State of the cobalt-manganese crust |
||
Air-dry |
As is (20 % moisture content) |
Water-saturated |
|
Ultimate tensile strength σt, MPa |
0.82 |
0.86 |
0.78 |
Ultimate compressive strength σc, MPa |
5.4 |
5.87 |
5.83 |
Ultimate shear stress (cohesion) C0, MPa |
1.35 |
1.45 |
1.02 |
Brittleness index Kbr |
6.5 |
6.7 |
7.45 |
Maximum shear resistance τmax, MPa |
8.6 |
9.42 |
10.14 |
The rock strength profile (Fig.7, a) represents plots for each state of the CMC samples consisting of interconnected tangents to the Mohr circles which radii are defined by the ultimate stress values for different types of failure.
The experimental tests on failure of the QMC by cutting were performed on the Zwick/Roell Z100 testing machine with a reference cutter. The results of these tests were used to plot the dependences of the cutting force on the compressive strain of the cobalt-manganese crust (Fig.7, b) while changing the chip thickness from 10 to 30 mm. Due to the high porosity and brittleness of the cobalt-manganese crust samples, it was not possible to achieve a repetitive series of chipping, the exception being a series of experiments with 20 mm chips, which was accompanied by several basic chippings, which is characteristic of the cutting process.
Experimental tests on the impact failure of the cobalt-manganese crust were carried out using a laboratory pendulum-type impact machine with a striker that was set at different angles and would strike the samples with different energy along or across the deposition layers of the cobalt-manganese crust. The depth of the striker penetration into the samples was recorded for each series of tests. Plots of the striker penetration depth versus the impact energy were made based on the test results (Fig.7, c).
Conclusion
The species composition and diversity of benthic biotic communities on the seafloor in the areas of the proposed mining of ferromanganese nodules, cobalt-manganese crust, and deep-water polymetallic sulphides are characteristic features of the observed ecosystems.
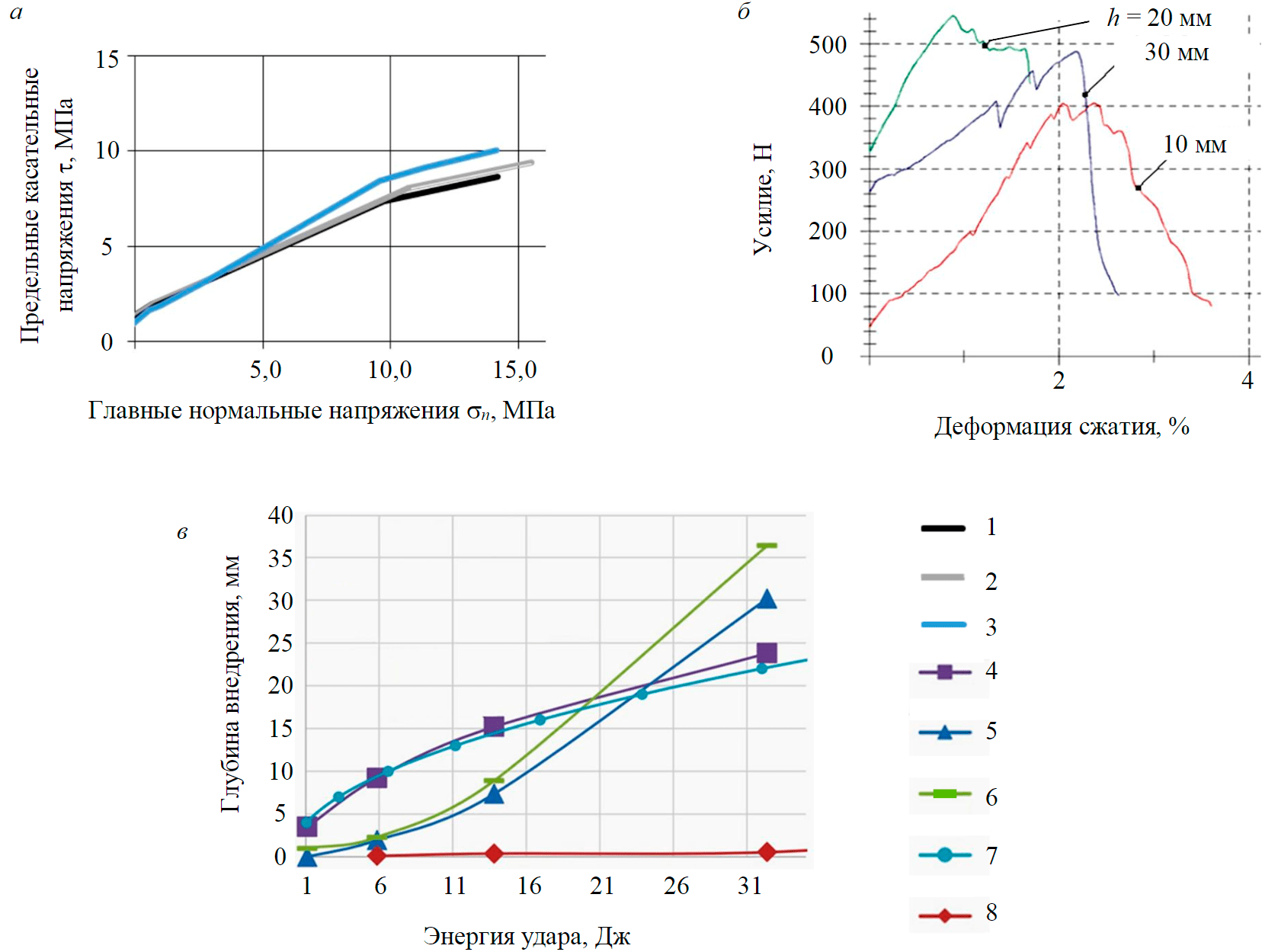
Fig.7. Experimental studies of CMC failure: by spherical indenters (a); by cutting using the Zwick/Roell Z100 testing machine (b); by impact forces using a pendulum-type impact machine (c) 1 – air-dried cobalt-manganese crust; 2 – as is (20 % moisture content); 3 – water-saturated cobalt-manganese crust; 4 – a strike across the cobalt-manganese crust bedding; 5 – a strike along the cobalt-manganese crust bedding; 6 – a strike along the bedding (together with the substrate); 7 – theoretical dependence; 8 – a strike against the substrate
The zone of ferromanganese nodules in the Russian Exploration Area in the Clarion-Clipperton deep-sea field is characterized with the absence of large concentrations of marine organisms and the morphology of the ore deposits, which is favourable for collecting the nodules from the seafloor.
Within the Magellan Seamounts, the cobalt-manganese crust is formed on the upper parts of the guyot slopes. Thus, operations to separate the cobalt-manganese crust from the bedrock are not expected to cause serious damage to the benthic fauna. However, the collateral effects of these operations associated with formation of sediment plumes and roiling of significant volumes of water may negatively affect the marine biota.
Three types of hydrothermal systems, differentiated in terms of their hydrothermal activity, the number, density and species diversity of marine life, have been identified within the deposits of deep-water polymetallic sulphides in the Russian Exploration Area (the Mid-Atlantic Ridge).
- Fields with limited present-day high-temperature hydrothermal activity and little (Ashadze-1) or no hydrothermal fauna (Ashadze-2). Fields of this type represent the majority of the studied
hydrothermal mineralization sites within the deposits of deep-water polymetallic sulphides in the Russian Exploration Area. When mining resources at such sites, it is necessary to use equipment that would maximize preservation of hydrothermal fauna and to restrict work within the impact zone of high-temperature hydrothermal vents. In addition to higher concentrations of living organisms around the vents, the negative impact of the high temperature acidic solutions on mining equipment should also be taken into account. - Active hydrothermal fields with abundant and unique fauna (the Logatchev field type) are characterized with high temperatures and aggressive behavior of the acidic fluids of the “black smokers”. Analysis of the occurrence conditions of solid minerals and specific features of the species composition and diversity of the hydrothermal biotic communities helps to identify typical hydrothermal fields in the Russian Exploration Area within the Mid-Atlantic Ridge. The greatest challenges the mechanisms face in mining these minerals are the presence of areas with increased and moderate hydrothermal activity (such as the Logatchev and the Ashadze-1 fields). Ore mining in these conditions is impossible without inflicting serious damage to the colonies of hydrothermal communities of living organisms close to the deep-water hydrothermal systems of the ocean. At the same time, it is possible to select inactive areas available for mining in the fields of low and medium activity (such as the Ashadze-1 and the Ashadze-2 fields).
- The absence of high-temperature discharge of ore-forming solutions and the hydrothermal fauna in inactive hydrothermal fields (such as the Krasnov and larger part of the Ashadze-2 fields) makes mining of the mineral resources most favorable using the developed machinery.
When mining the considered types of solid minerals, it should be taken into account that a certain amount of benthic organisms (especially microbiota) is always present even in relatively “deserted” areas of the ocean floor. In selecting the technology and tools to mechanize the mining processes, it is therefore very important to choose specific designs of effectors that would minimize the mechanical impacts on both the seabed surface and the underlying rocks and benthic waters. It is worth noting that the contrasting occurrence conditions and the composition of benthic ecosystems do not allow creating a universal design of the mining equipment that would be capable of preserving the deep water inhabitants of all of the above types of solid mineral deposits.
Analyzing features of the developed devices in terms of the environmental aspects (roiling, mechanical impact on the sea floor) makes the devices with suction chambers and a grip arm walking gear top priorities.For the cobalt-manganese crust that is found in particularly difficult conditions, the technology developed by Saint Petersburg Mining University stands out as the most efficient since it features collecting units with grip arms with integrated clamshell-type effectors and impactors. In order to create a feasible design for mining deep-sea ferromanganese nodules or cobalt-manganese crust with a significant variation in average nodule sizes (50-250 mm) or lumps of broken-off cobalt-manganese crust (in the presence of abundant and unique forms of flora and fauna), it is proposed to use the concept developed at Saint Petersburg Mining University. It is presented as a specific design of a deep-sea bottom collecting unit with enhanced environmental performance. Correct application of this concept with fine-tuning of the walking gear and selection of rational design parameters for both the collecting unit and the effector will ensure that the mechanical impact on the bottom areas is minimized.
The following designs have been proposed and patented to secure efficient collection of deep-water cobalt-manganese crust: a concept of ferromanganese nodules and cobalt-manganese crust collection; a walking-type unit for cobalt-manganese crust collection and a clamshell-type effector with an integrated shearing tool, i.e. an impactor. The performed experimental testing of the cobalt-manganese crust samples in terms of their collection efficiency with a clamshell-type effector with an integrated impactor has shown that it is possible:
- to translate the obtained values of physical and mechanical properties of the available cobalt-manganese crust samples to the mineral mass in actual mining and geological conditions;
- to penetrate into the upper (less strong) layers of the cobalt-manganese crust using a clamshell-type bucket with an axial force of 300-600 N;
- to intensify penetration into the middle layers of the cobalt-manganese crust, and splitting minerals pieces off the substrate by means of impactors integrated into the clamshell jaws using the impact energy of up to 30 J.
References
- Sudarikov C.M., Zmievskii M.V. Geochemistry of ore-forming hydrothermal fluids of the world ocean. Journal of Mining Institute. 2015. Vol. 215, p. 5-15 (in Russian).
- Sudarikov S.M. Modeling of Geochemical Processes in the Submarine Discharge Zone of Hydrothermal Solutions. Journal of Mining Institute. 2017. Vol. 225, p. 284-291. DOI: 10.18454/PMI.2017.3.284
- Van Dover C.L., Smith C.R., Ardron J. et al. Environmental management of deep-sea chemosynthetic ecosystems: justification of and considerations for a spatially-based approach. ISA Technical study International Seabed Authority. Kingston: International Seabed Authority, 2011. N 9, p. 90.
- Yungmeister D.A., Sudarikov S.M., Kireev K.A. Feasibility of Type of Deep-Water Technologies for the Extraction of Marine Ferro-Manganese Nodules. Journal of Mining Institute. 2019. Vol. 235, p. 88-95. DOI: 10.31897/PMI.2019.1.88
- Yungmeister D.A., Smirnov D.V., Verzhanskii A.P., Isaev A.I. Machines and equipment for ferromanganese nodule mining. St. Petersburg: Politekhnika-servis, 2015, p. 135 (in Russian).
- Serzhan S.L. Determining the rational immersion depth of a mining complex capsule for underwater mining of ferromanganese nodules. Procedia Engineering. 2016. Vol. 150, p. 924-929. DOI: 10.1016/j.proeng.2016.07.063
- Melnikov M.E., Pletnev S.P. Age and Formation Conditions of the Co-Rich Manganese Crust on Guyots of the Magellan Seamounts. Lithology and Mineral Resources. 2013. Vol. 48. N 1, p. 1-13. DOI: 10.1134/S0024490212050057
- Liu C., Massey M.S., Latta D.E. et al. Fe(II)-induced transformation of iron minerals in soil ferromanganese nodules. Chemical geology. 2021. Vol. 559, p. 119901. DOI: 10.1016/j.chemgeo.2020.119901
- Shulga N.A.Characteristics of Alkanes in Ferromanganese Nodules of the Clarion-Clipperton Fracture Zone. Oceanology. 2018. Vol. 58. Iss. 5, p. 672-678.
- Kamenskaya O.E., Melnik V.F., Gooday A.J. Giant Protists (Xenophyophores and Komokiaceans) from the Clarion–Clipperton Ferromanganese Nodule field (Eastern Pacific). Journal of General Biology. Vol. 73. N 5, p. 377-388 (in Russian).
- Durden J.M., Bett B.J., Ruhl H.A. The hemisessile lifestyle and feeding strategies of Iosactis vagabunda (Actiniaria, Iosactiidae), a dominant megafaunal species of the Porcupine Abyssal Plain. Deep-Sea Research. Part I: Oceanographic Research Papers. 2015. Vol. 102, p. 72-77. DOI: 10.1016/j.dsr.2015.04.010
- Ramirez Llodra E., Billett D.S.M.Deep-sea ecosystems: pristine biodiversity reservoir and technological challenges. The Exploration of marine biodiversity: scientific and technological challenges. Bilbao, Spain: Fundacion BBVA, 2006, p. 63-92.
- Gollner S., Kaiser S., Menzel L. et al. Resilience of benthic deep-sea fauna to mining activities. Marine Environmental Research. 2017. Vol. 129, p. 76-101. DOI: 10.1016/j.marenvres.2017.04.010
- Miljutin D., Miljutina M., Arbizu P., Galéron J. Deep-sea nematode assemblage has not recovered 26 years after experimental mining of polymetallic nodules (ClarionClipperton Fracture Zone, Tropical Eastern Pacific). Deep-Sea Research. Part I: Oceanographic Research Papers. 2011. Vol. 58. Iss. 8, p. 885-897. DOI: 10.1016/j.dsr.2011.06.003
- Hein J.R., Koschinsky A. Deep-Ocean Ferromanganese Crusts and Nodules. Treatise on Geochemistry (Second Edition). Amsterdam, Netherlands: Elsevier, 2014, p. 273-291. DOI: 10.1016/B978-0-08-095975-7.09879-X
- Melnikov M.E. Cobalt-bearing manganese crusts of seamounts. Current state of the issue. VII Vserossiiskoe litologicheskoe soveshchanie “Osadochnye basseiny, sedimentatsionnye i postsedimentatsionnye protsessy v geologicheskoi istorii”, 28-31 oktyabrya 2013, Novosibirsk, Rossiya; Institut neftegazovoi geologii i geofiziki im. A.A.Trofimuka. 2013. Vol. 2, p. 264-268 (in Russian).
- Garrigue C., Geyer Y., Kennedy A.S., Zerbeni A.N. Satellite tracking reveals novel migratory patterns and the importance of seamounts for endangered South Pacific humpback whales. Royal Society Open Science. 2015. Vol. 2. Iss. 11. N 150489. DOI: 10.1098/rsos.150489
- Watling L., Auster P. Seamounts on the High Seas Should Be Managed as Vulnerable Marine Ecosystems. Frontiers in Marine Science. 2017. Vol. 14, p. 14. DOI: 10.3389/fmars.2017.0001
- Anantharaman K., Dreier J.A., Dick G.J. Metagenomic resolution of microbial functions in deep-sea hydrothermal plumes across the Eastern Lau Spreading Center. The ISME Journal. 2016. Vol. 10, p. 225-239. DOI: 10.1038/ismej.2015.81
- Petersen J.M., Zielinski F.U., Pape T. et al. Hydrogen is an energy source for hydrothermal vent symbioses. Nature. 2011. Vol. 476, p. 176-180. DOI: 10.1038/nature10325
- Rivers A.R., Sharma S., Tringe S.G. et al. Transcriptional response of bathypelagic marine bacterioplankton to the Deepwater Horizon oil spill. The ISME Journal. 2013. Vol. 7, p. 2315-2329. DOI: 10.1038/ismej.2013.129
- Baker E.T. Hydrothermal plumes. Encyclopedia of Marine Geosciences. Encyclopedia of Earth Sciences Series. Springer, 2016, p. 994. DOI: 10.1007/978-94-007-6238-1_16
- Baker E.T., Resign J.A., Haymon R.M. et al. How many vent fields? New estimates of vent field populations on ocean ridges from precise mapping of hydrothermal discharge locations. Earth and Planetary Science Letters. 2016. Vol. 449, p. 186-196. DOI: 10.1016/j.epsl.2016.05.031
- Beaulieu S.E., Baker E.T., German C.R. Where are the undiscovered hydrothermal vents on oceanic spreading ridges? Deep-Sea Research. Part II: Topical Studies in Oceanography. 2015. Vol. 121, p. 202-212. DOI: 10.1016/j.dsr2.2015.05.001
- Boschen R.E., Rowden A.A., Clark M.R. et al. Megabenthic assemblage structure on three New Zealand seamounts: implications for seafloor massive sulfide mining. Marine ecology progress series. 2015. Vol. 523, p. 1-14. DOI: 10.3354/meps11239
- Fouquet Y., Cherkashov G., Charlou J.L. et al. Serpentine cruise – ultramafic hosted hydrothermal deposits on the Mid-Atlantic Ridge: First submersible studies on Ashadze 1 and 2, Logatchev 2 and Krasnov vent fields. InterRidge News. 2008. Vol. 17, p. 15-19.
- Charlou J.-L., Donval J.-P., Konn C. et al. High hydrogen and abiotic hydrocarbons from new ultramafic hydrothermal sites between 12°N and 15° N on the Mid-Atlantic Ridge. Results of the Serpentine cruise (March 2007). Geophysical Monograph Series. 2007. Vol. 188, p. 265-296. DOI: 10.1029/2008GM000752
- Fabri M-C., Bargain A., Briand P. et al. The hydrothermal vent community of a new deep-sea field, Ashadze-1, 12°58′ N on the Mid-Atlantic Ridge. Journal of the Marine Biological Association of the United Kingdom. 2010. Vol. 91. Iss. 1, p. 1-13. DOI: 10.1017/S0025315410000731
- Fouquet Y., Cherkashov G., Charlou J. et al. Diversity of ultramafic hosted hydrothermal deposits on the mid Atlantic ridge: first submersible studies on Ashadze, Logatchev 2 and Krasnov Vent Fields during the Serpentine Cruise. AGU Fall Meeting Abstracts. 2007. Vol. 2007. N T51F-03.
- Sudarikov S.M., Roumiantsev A.B. Structure of hydrothermal plumes at the Logatchev vent field, 14°45'N, Mid-Atlantic Ridge: evidence from geochemical and geophysical data. Journal of Volcanology and Geothermal Research. 2000. Vol. 101, p. 245-252. DOI: 10.1016/S0377-0273(00)00174-8
- Petersen S., Kuhn K., Kuhn T. et al. The geological setting of the ultramafic-hosted Logatchev hydrothermal field (14°45'N, Mid-Atlantic Ridge) and its influence on massive sulfide formation. Lithos. 2009. Vol. 112, p. 40-56. DOI: 10.1016/j.lithos.2009.02.008
- Samarina T.E. Specific features in geochemistry of the Irina-2 hydrothermal ecosystem. Journal of Mining Institute. 2006. Vol. 167 (2), p. 46-48 (in Russian).
- Cherkashov G., Poroshina I., Stepanova T. et al. Seafloor massive sulfides from the Northern Equatorial Mid-Atlantic Ridge: new discoveries and perspectives. Marine Georesources & Geotechnology. 2010. Vol. 28. Iss. 3, p. 222-239. DOI: 10.1080/1064119X.2010.483308
- Lipton I., Gleeson E., Munro P. Preliminary Economic Assessment of the Solwara Project, Bismark Sea. Technical report compiled under NI 43-101. Toronto, Canada: PNG – Nautilus Minerals Niugini, 2018, p. 242.
- Ishiguro S., Yamauchi Y., Odaka H., Akiyama S. Development of Mining Element Engineering Test Machine for Operating in Seafloor Hydrothermal Deposits. Mitsubishi Heavy Industries Technical Review. 2013. Vol. 50. N 2, p. 21-26.
- Ishiguro S., Masuda M., Komatsu M. et al. Development of the Pilot System for Test of Excavating and Ore lifting of Seafloor Polymetallic Sulfides. Mitsubishi Heavy Industries Technical Review. 2018. Vol. 55. N 3, p. 1-7.
- Sharma R. Deep-Sea Mining: Current status and future considerations. Deep-Sea Mining. Resource potential, technical and environmental considerations. 2017, p. 3-21. DOI: 10.1007/978-3-319-52557-0_1
- Tetsuo Y. Fundamental geotechnical consideration for design of deep-sea mining systems. Deep-Sea Mining. Resource potential, technical and environmental considerations. 2017, p. 259-295. DOI: 10.1007/978-3-319-52557-0_9
- Atmanand M.A., Ramadass G.A. Concepts of deep-sea mining technologies. Deep-Sea Mining. Resource potential, technical and environmental considerations. 2017, p. 295-341. DOI: 10.1007/978-3-319-52557-0_10
- 40. Serzhan S.L., Medvedkov V.I. Influence of the depth of immersion of a capsule with pulp pumping equipment on the efficiency of mining of mineral resources by the offshore mining complex. Mining equipment and electrical engineering. 2016. N 3, p. 34-42 (in Russian).
- Iungmeister D.A., Korolev R.I., Serzhan S.L. et al. Materials of devices and equipment for deep-sea mining of manganese resources. High-Tech and Innovations in Research and Manufacturing (HIRM-2020) 28 February 2020, Siberia, Russia. Journal of Physics: Conference Series, 2020. Vol. 1582. N 012098. DOI: 10.1088/1742-6596/1582/1/012098
- Yungmeister D.A., Isaev A.P., Serzhan S.L. Patent RU 186415 U1. A bottom mining unit for collection of ferromanganese nodules from the seafloor. Publ. 21.01.2019. Bul. N 4 (in Russian).
- Yungmeister D.A., Isaev A.P., Serzhan S.L. et al. Patent RU 193043 U1. A device to collect ferromanganese nodules from the seafloor. Publ. 11.10.2019. Bul. N 29 (in Russian).
- Yungmeister D.A., Korolev R.I., Serzhan S.L., Urazbakhtin R.Yu. Patent RU 203596 U1. A device to collect cobalt-manganese crust from the seafloor. Publ. 13.04.2021. Bul. N 11 (in Russian).
- Korshunov V.A., Solomoychenko D.A., Bazhukov A.A. Strength estimation of fractured rock using compression – a specimen with spherical indenters. ISRM European Rock Mechanics Symposium – EUROCK 2018, 22-26 May 2018, St. Petersburg, Russia. OnePetro, 2018. N ISRM-EUROCK-2018-034.