Genetic geological model of diamond-bearing fluid magmatic system
- 1 — Ph.D., Dr.Sci. Head of Department Empress Catherine II Saint Petersburg Mining University ▪ Orcid
- 2 — Ph.D., Dr.Sci. Leading Researcher Empress Catherine II Saint Petersburg Mining University ▪ Orcid ▪ Elibrary ▪ Scopus
- 3 — Ph.D. Leading Researcher Empress Catherine II Saint Petersburg Mining University ▪ Orcid
- 4 — Ph.D. Associate Professor Empress Catherine II Saint Petersburg Mining University ▪ Orcid ▪ Elibrary ▪ Scopus ▪ ResearcherID
- 5 — Ph.D. Associate Professor Empress Catherine II Saint Petersburg Mining University ▪ Orcid
Abstract
The article proposes a genetic geological model of diamond deposit formation associated with kimberlites and lamproites. It is based on the synthesis of published data on diamond-bearing kimberlite systems and an original study of the ontogenetic features of diamond crystals. Deep diamond crystallization, its upward transportation and subsequent concentration in near-surface kimberlite-lamproite bodies and other rocks, including those brought to the surface by high-amplitude uplifts of crystalline basement rocks, are combined in a single system. An assumption is made about the primary sources of the Anabar placer diamonds. The possibility of hydrocarbon generation at mantle levels corresponding to diamond formation areas and their transportation to the upper crustal zones by a mechanism similar to the mantle-crust migration of diamond-bearing fluidized magmas is shown. The high rate of their upward movement allows transportation to the surface without significant loss as a result of dissolution in melts and sorption on the surface of mineral phases. The significant role of fluid dynamics at all stages of this system is noted.
Funding
The article was preparedunder the state assignment FSRW-2024-0008 “Investigation of thermodynamic processes of the Earth with regard to the genesis of hydrocarbons at great depths”.
Introduction
Great interest in diamonds and their formation conditions is associated not only with the high value of this mineral as a jewellery and technical raw material, but also with the fact that it is a source of unique information about the substance and thermodynamic conditions in the mantle depths inaccessible for direct study. A correct understanding of the conditions for the diamond deposit formation allows for effective forecasting and exploration and increases the effectiveness of mineral resource management policies [1]. Diamond, mantle xenoliths, kimberlites, and lamproites are mantle matter fragments accessible for study, but only diamond can deliver this matter to the Earth’s surface in its original form. A significant interval of diamond formation depths (120-600 km from the Earth’s surface) [2] allows for an analysis of the fluid and mineral matter characteristics at these depths. For example, the study of fluid inclusions in diamonds revealed the presence of methane and heavy hydrocarbons, which proved the fundamental possibility of generating hydrocarbon fluids in mantle conditions [3-5].
Due to the great depths of diamond formation and their concentration in near-surface kimberlite bodies (dikes, sills, pipes), the question arises about the mechanism of their transportation from the mantle depths to the surface. In the proposed genetic geological model of the diamond-bearing fluid magmatic system, the essential elements are considered and structured: deep crystallization of diamond, its ascending mantle-crust transportation, and subsequent concentration in near-surface kimberlite and lamproite bodies.
Conceptual model of diamond-bearing fluid magmatic system
The formation system of primary diamond deposits associated with kimberlites and lamproites incorporates three major subsystems: crystallization of diamond and its transition to a mobile form; mantle-crust migration of diamond; diamond concentration in a near-surface kimberlite (lamproite) body (dike, sill, pipe).
This system is hard to study due to its large vertical extent (to 600-750 km), encompassing the levels of the sublithospheric mantle, lithospheric mantle, and continental crust, a wide range of thermodynamic parameters of its functioning in this depth interval (Т = 0-1500 °С, Р = 10–4-8 GPa), and limitations in obtaining direct information about its material composition [6, 7]. A conceptual model of the system is shown in Fig.1.
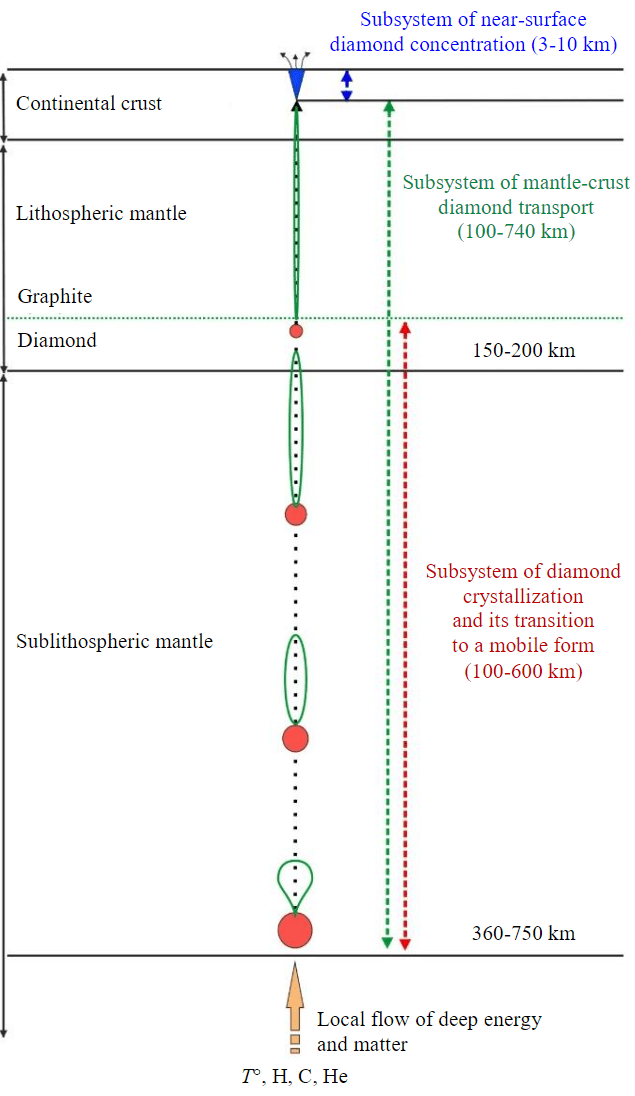
Fig.1. Diamond deposit formation system
The identified subsystems are studied to varying degrees. The most accessible for study upper level is characterized in more detail – the subsystem of near-surface diamond concentration, represented by diamond-bearing kimberlite and lamproite pipes, sills, and dikes. These geological formations are studied by geophysical methods, exposed by mine workings, kimberlites and mantle xenoliths and diamonds extracted from them are studied in detail. The extensive information obtained about the morphological features of kimberlite bodies, structural and textural features of kimberlites, their mineral and chemical composition allowed various researchers to come to similar genetic geological models of diamond-bearing kimberlite bodies formation [8-10].
The diamond formation subsystem is studied to a lesser extent, but in sufficient detail. Despite the exceptionally large depths of its functioning, inaccessible for direct observation, the study of mantle xenoliths, diamonds, as well as a significant volume of experimental research on diamond growth in various environments made it possible to come closer to understanding their formation conditions. For a long time, the representativeness of the data obtained in diamond study was limited by the availability of only small crystals for study. In recent years, within the framework of the Gemological Institute of America (GIA) activities, the largest diamonds and brilliants have become available for research using non-destructive methods, and their fragments remaining after cutting were studied using destructive methods. As a result, exceptionally important data were obtained characterizing the diamond formation conditions in the sublithospheric mantle at depths of 600 km or more [11].
The least studied subsystem is the mantle-crust diamond transportation, which transports diamonds from their crystallization level in the mantle (120-600 km) to the Earth’s surface, where they are concentrated in kimberlite or lamproite bodies. The key condition that this subsystem must meet is the preservation of diamond, which crosses the thermodynamic stability boundary during upward transportation and can turn into graphite. The difficulty also lies in the fact that models developed for the upward magma movement during the volcanic systems formation usually assume a hydrodynamic connection between the intermediate magma chamber and the surface [12, 13]. This is acceptable when the intermediate magma chamber is at a depth of a few km but is unavailable when diamond-bearing kimberlite magma originates at a depth of 120-600 km from the Earth’s surface.
The aim of the study is to develop a genetic geological model of diamond deposits associated with kimberlites and lamproites, which combines in a single system the deep crystallization of diamond, its upward transportation, and subsequent concentration in near-surface kimberlite-lamproite bodies. The issues of the formation of deep hydrocarbons and possible mechanisms of their transportation to the near-surface zones of the Earth’s crust, where they can participate in the hydrocarbon deposits formation, are considered.
Subsystem of diamond crystallization and its transition to a mobile form
Based on the study of inclusions in diamonds, assumptions are made about their possible formation environments. Direct growth of diamonds could take place in a liquid (fluid) environment of various compositions (silicate, sulphide, carbonate, metallic) [7, 14, 15]. Based on the results of studying syngenetic inclusions in diamonds, the silicate-carbonate environment is considered one of the most probable for diamond growth [16, 17], which is confirmed experimentally as well [18].
The limited occurrence of metallic inclusions in diamonds did not allow metal melts to be considered as the predominant alternative of natural diamond-forming environment. However, a recent study of high-quality Cullinan-like diamonds of the CLIPPIR type (Cullinan-like, Large, Inclusion-Poor, Pure, Irregular and Resorbed) allowed to reveal new features of their formation [7, 11]: tiny iron-nickel metallic inclusions surrounded by a fluid shell consisting of methane and hydrogen. A comprehensive study of these diamonds enabled to assume their growth in a melt of nickel-bearing iron (containing carbon and hydrogen in dissolved form) under sharply reducing conditions of the sublithospheric mantle at depths of about 600 km. Fluid methane-hydrogen shells were found around metal inclusions in synthetic diamonds as well [19]. It is suggested [11] that at depths of about 250 km and below, metallic iron may be one of the stable phases in mantle rocks, and the lower mantle may contain approximately 1 wt.% of iron-rich metallic melt [20]. Iron may arise as a result of a disproportionation reaction in which the valence electrons in existing Fe2+ atoms are redistributed by the reaction
where Fe3+ is part of the silicates, and Fe0 is released into its own metallic phase [19].
Experimental studies confirmed the reality of native metals (Fe, Ni, Co) formation in the Earth’s crust during hydrogen interaction with basaltic melts, even under the hypabyssal facies parameters. It was shown for the first time that the metal-silicate liquation in basaltic melts during their interaction with hydrogen can occur at real magma temperatures in nature (≤ 1250 °C), which are significantly lower than the melting temperatures of iron and its alloys with nickel and cobalt. Н2О content in the basaltic melt increases significantly, which is associated with the fact that all reactions of iron oxide reduction with hydrogen lead to water formation [21].
The development of ideas about the diamond crystal formation conditions, new data on the diversity of their thermal history, morphology and anatomy [22], inclusion composition [23] and defect-impurity composition [24] enable distinguishing several groups of crystals that grew under different conditions and, probably, in different environments. Each primary deposit contains crystals of all groups [25], but a special aggregate are type IIa nitrogen-free diamonds, which include crystals assigned to the CLIPPIR group [11]. These crystals without zonal and sectoral inhomogeneities, with a polycentric surface structure, contain iron-nickel metallic inclusions.
A study of the distribution features of hydrogen and nitrogen defects in diamond crystals revealed an important pattern: an increase in temperature at a certain stage of diamond crystal growth is accompanied by an increase in hydrogen concentration in it [16]. Therefore, it can be assumed that hydrogen was the main supplier of deep energy necessary for the diamond-forming system functioning.
Summarizing the brief analysis of the diamond crystallization conditions in the mantle, we can identify the primary points for understanding the state of the diamond formation environment and its thermodynamic parameters.
Diamonds crystallized in a heterogeneous medium containing solid, liquid, and possibly gas phases. The liquid phase was probably also heterogeneous and had a silicate-carbonate or silicate-metal composition. The gas components, primarily methane and hydrogen, were in a dissolved state, but could also be partially present as a gas phase.
Hydrogen solubility in silicate melts under thermodynamic parameters corresponding to diamond formation is insignificant and, according to experimental results, is about ~7∙10−3 wt.% [21, 26], while for metallic iron melt the solubility increases by an order of magnitude and is 4∙10−2 wt.% [27, 28]. This determines the preferential accumulation of hydrogen in iron melt fragments as they pass through the silicate melt.
Methane can be considered as the main source of carbon for diamond growth. During the CLIPPIR-type diamonds crystallization, in the iron-nickel melt shell metallic iron may react with methane with free carbon formation [19]. Carbon will be concentrated mainly in the iron-nickel melt due to its greater solubility in it compared to the silicate melt. The carbon-saturated iron-nickel melt in this case represents a diamond-forming medium, the captured fragments of which were detected during the CLIPPIR-type diamonds study. The outline of this process is shown in Fig.2.
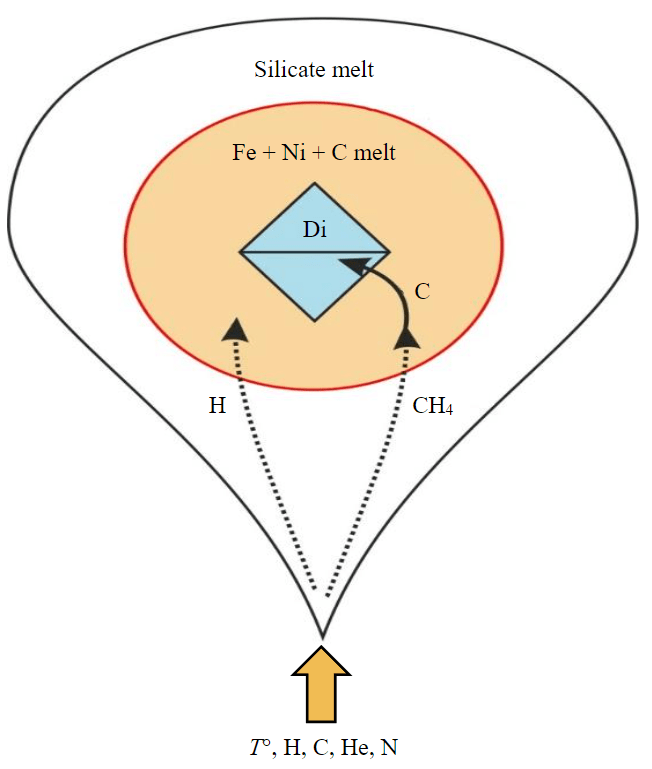
Fig.2. Diamond formation subsystem in iron-nickel melt
Equilibrium temperatures and pressures determined for diamonds by various methods are in the range of 900-1300 °C and 4.5-6.5 GPa, respectively [29]. It was experimentally shown that seeded diamond growth is possible starting from a temperature of 1400 °C at 7.5 GPa and 1520 °C at 6.3 GPa. Homogeneous diamond nucleation in kimberlite melt requires higher P-T-parameters (1570 °C at 7.5 GPa) [30]. Consequently, thermodynamic parameters in the lithospheric and sublithospheric mantle in the depth range of 120-750 km determine the possibility of diamond crystal growth in silicate-carbonate and iron-nickel melts and enable their movement in the corresponding fluid-saturated liquid environments.
The composition of fluid components, which are transported from the mantle depths to the surface in the form of inclusions in diamond, is interesting. Even early studies conducted by S.I.Melton and A.A.Giardini [4, 5, 31] and confirmed later [32], made it possible to detect Н2, Н2О, СО, СО2, СН4, N2, as well as O2, Ar, and heavy hydrocarbons in inclusions. The possibility of hydrocarbon formation due to the reaction of carbon from various sources (carbonates, graphite, diamond, amorphous carbon) and hydrogen [33], generated by Fe0 or Fe+2O reaction with water, was experimentally proven.
Important data were obtained when studying interaction in the carbonate (CaCO3, MgCO3, Ca(Fe0.5Mg0.5)(CO3)2) – wustite (FeO) or Fe0 – H2O system [34-36]. We found that such interaction provides the formation of a mixture of light alkanes with predominating CH4 at the P-T-parameters of the upper mantle. Moreover, a significant amount of oxygen-containing organic substances (C9-C10 aldehydes) and heavy alkanes (C12-C16) can arise in a water-rich fluid [36]. Experimental studies showed that in mantle conditions, the probability of generating increasingly heavier hydrocarbons grows with depth [37]. Consequently, theoretical estimations, experimental studies, and analysis of fluid inclusions in diamonds indicate the existence of hydrocarbon compounds in mantle conditions.
Mechanisms of diamond transportation from the mantle region of their crystallization to the final near-surface diamond-bearing kimberlite body
The main regulating moment in diamond transportation from the crystallization region to the near-surface kimberlite or lamproite body are their preservation conditions. On the ascending trajectory, the diamond crosses its stability boundary and can transform into graphite. The rate of this reaction depends on the temperature, pressure, and composition of the medium in which the diamond moves to the Earth’s surface. A significant rate of diamond transformation into graphite is achieved only at a temperature above 1000 °C, which corresponds to a depth of more than 40 km. Consequently, the ascending diamond transportation from its formation place to the depths ensuring its preservation must proceed either at a high rate or in a medium preserving its stability conditions over a significant interval of its movement. A combination of these conditions is also possible.
Conclusions about the rate of kimberlite magma ascent are based on various kinetic constraints and suggest typical rates in the range of 10-30 m/s in deep-seated environments [38, 39]. The dynamics of this process can be refined by studying the material and ontogenetic diamond properties. For example, placer diamonds in the Zimmi area in West Africa have a low degree of nitrogen aggregation with a predominance of C-centres. This feature is explained by the fact that after the growth completion, the diamonds were quickly moved to depths within which the temperatures are no longer sufficient for nitrogen aggregation [40].
The main models of diamond transportation from the crystallization region to the near-surface parts of the Earth’s crust can be divided into two types. The first assumes a hydrodynamic connection between the areas of formation and final accumulation of diamonds. The second type is based on the idea of local portions of diamond-bearing kimberlite magma, which are separated from the original diamond-forming magma centre and autonomously move to the surface. Since researchers have no information about the substance along the route of the kimberlite melt movement, when choosing the most preferable option, it is necessary to consider the morphological features, the internal structure of kimberlite bodies, and the specifics of the diamonds contained in them.
The key feature of kimberlite pipes, the main repositories of deep diamonds, which are volcanic edifices [10], is the limited volume of kimberlite material contained in them. Unlike ordinary volcanoes, kimberlite analogues never form large volcanic structures. On weakly eroded kimberlite bodies (the Orapa and Mwadui pipes), only low peripheral tuff rings are formed in the near-surface part, and crater lake deposits are noted in the crater part. The studies of the youngest Holocene kimberlite bodies discovered in Tanzania, which are preserved almost in their original form, are very indicative [41]. They are low volcanic structures, the height of which, even considering the eroded part, did not exceed 100 m, composed of pyroclastic material with a small volume of lava. These features are explained by the kimberlite diatreme formation as a result of local, limited-volume portion of fluidized kimberlite magma entering into the near-surface zone of the Earth’s crust.
Existing models of kimberlite ascent assume the upward movement of a local vertically limited fracture filled with magma rich in volatile components through the mantle lithosphere. Gas emission and liquid phase expansion in the migrating fracture creates pressure gradients of more than 70 MPa [42], which allows it to rise at a high rate. Such a discrete-pulse mechanism is considered in detail in [43-45]. The work [43] analyses the differentiation processes in the moving mantle matter portion represented by a mixture of fluidized silicate melt, mantle xenoliths, and diamonds. This heterogeneous fluid portion can autonomously move upward due to half-opening of the upper fracture wedge-out with an increase in volume and a parallel drop in pressure in the system, leading to collapse in the lower fracture wedge-out area [45]. According to the proposed mechanism, a decrease in pressure in the fracture cavity leads to partial melt degassing and creation of a pseudofluidized suspension rich in solid particles. Two oppositely directed forces act on the solid fragments in the fluidized silicate melt filling the fracture: the downward gravity force and the upward force of the ascending flow of gas bubbles from the degassed magma. Active interaction between the solid particles promotes the mechanical grinding of mantle xenoliths and xenocrystals, causing a change in their shape and surface character. The mantle load (xenocrystals and xenoliths) continuously deposits through the melt column, accumulating in the lower fracture wedge-out area, along the trace of which a tail rich in crystals and xenoliths is formed [43].
Key aspects of the model under consideration:
- The origin of diamond-bearing mantle melt occurs in an area of increased plasticity, which suggests a close to spherical shape of the initial melt portion.
- When entering into more brittle rocks during the upward movement, the initial melt portion will acquire an increasingly flattened and vertically extended shape, which contributes to an increase in the rate of its rise due to an increase in the pressure gradient between its lower and upper wedge-out.
- The ability of a melt portion to move upward depends on the excess pressure of the fluid components (H2O, CO2, H2, CH4, etc.) dissolved in the melt. This model is implemented only under the condition of a significantly higher pressure in the ascending melt portion compared to the lithostatic pressure, which assumes the preservation of the thermodynamic parameters of diamond stability to higher levels during its upward movement. The upward movement of a local intra-fracture melt portion can cease when the accumulated energy is exhausted and resume with its additional supply in the form of a deep fluid flow.
- While moving upward, phase differentiation of this heterogeneous system will occur with the gas phase movement to the upper part and the solid phases deposition in the lower wedge-out area. The result of the solid phases capture during the fracture collapse in the lower wedge-out area is especially interesting. According to R.K.Brett, this crystal-rich tail (relict dike) forms a semblance of a hypabyssal intrusion [43]. However, given the extremely high rate of fracture advancement in such a mechanism (to 20-90 m/s) [46], it is difficult to assume the accumulation of a large volume of solid phase; rather, it will be a chain of mantle xenoliths, their fragments, and what is essential, diamonds captured during the fracture collapse. In fact, this is a new type of diamond concentrations, which is unlikely to be of commercial value due to the low probability of getting to the modern surface and low diamond concentration, but it can be assumed to outcrop to the erosional truncation in the most eroded crystalline basement fragments, where these formations can become the primary sources of placers. Probably, such formations were suppliers of diamonds to the placers of the eastern slope of the Anabar Shield, the primary sources of which have not yet been discovered.
- The mechanism of discrete fracture movement under consideration is similar to that of hydraulic fracturing, which is widely used to activate oil recovery. This technology involves the use of proppant – solid granules that prevent the complete closure of hydraulic fractures. Solid fragments of mantle matter captured as the hosting fracture moves upward can also function as proppant, maintaining permeability for deep fluid components within the trajectory of the moving fracture trace. Additional mantle fluid portions can move along this permeable structure, supporting its upward movement. The outline of the discrete-pulse system for transporting a diamond-bearing melt portion is shown in Fig. 1, and individual stages of its movement are reflected in Fig.3.
The different contribution of tectonics and fluid dynamics to the upward transportation of local portions of diamond-bearing melt should manifest itself at different depth levels. In the sublithospheric mantle, with a relatively high proportion of the liquid phase, the leading role in the movement of fluid-saturated fragments of diamond-bearing melt will be played by fluid dynamics. During the upward movement and segregation of its drop-shaped isolations, larger accumulations of fluidized melt will arise, the movement of which can be supported by the influx of additional gas phase portions. During the transition along the upwelling into the solid lithospheric mantle medium, a fracture cavity will be formed by a mechanism close to hydraulic fracturing. At this stage, it is important to emphasize the primacy of fluid dynamic processes that use the already existing heterogeneities in rocks (including tectonic fractures) to generate a fracture cavity filled with diamond-bearing fluidized magma. With further autonomous upward movement, it can conjugate with other previously formed tectonic fractures or form them by the hydraulic fracturing mechanism, using local heterogeneities in rocks of any origin.
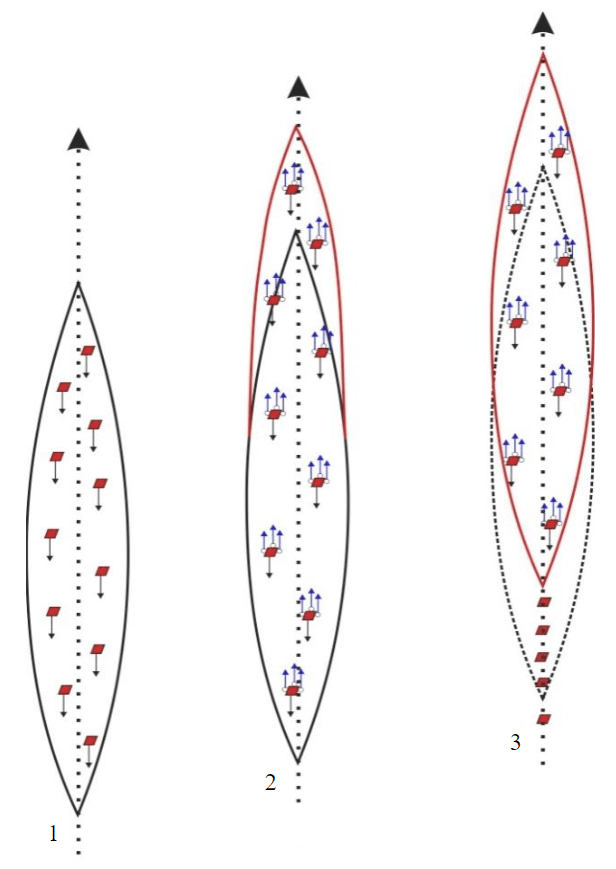
Fig.3. Scheme of discrete-pulse system of ascending diamond-bearing fluidized melt migration
1 – initial state of a melt-filled fracture with crystalline fragments; 2 – fracture half-opening in the upper pinch-out area with an increase in the fracture cavity volume and magma degassing; 3 – fracture collapse in the lower pinch-out area with the crystalline fragments capture along its movement route
Diamond concentration in near-surface kimberlite bodies
The kimberlite pipe formation mechanism was examined in numerous publications [9, 10, 47]. V.A.Milashev identifies two main stages in this process: the pipe-shaped cavity stage, which develops from the Earth’s surface inward, and the stage of its filling with magmatic material, starting from depth to the surface [10].
Most researchers studying kimberlite pipes concluded that diatremes are formed with the participation of magmatic melts, fluids, and gases [44, 48]. Opinions differ on the significance of individual phases in cavity formation and pipe development. However, it is difficult to deny that a necessary condition for these processes is the presence of a fluid flow, which, after separation from the kimberlite magma, determines the fluid dynamic regime during the explosive phase of kimberlite bodies formation, and, passing through the magma, acts as a transmagmatic fluid. According to [46, 48], the kimberlite fluid was a liquid of predominantly carbonate-magnesia composition, enriched in water and carbon dioxide, containing a suspended solid phase.
The functions of this fluid are varied: active heat and mass transfer in the kimberlite magmatic system from the lower to the upper levels; the main driving force for the transfer of mantle xenoliths, diamonds, and other solid fragments from various levels of the kimberlite system to the surface; development of a pipe-shaped cavity hosting a kimberlite body; intra-pipe differentiation of kimberlite magma; formation of automagmatic metasomatites.
It is necessary to note a fundamental aspect in the mechanism of kimberlite and lamproite bodies formation that has not been sufficiently reflected in publications. When a diamond-bearing fluid magmatic system develops, the silicate melt in it after rising to the root part level of the pipe behaves passively. The main processes of pipe-shaped cavities formation and the specificity of their infilling mechanism are regulated by the fluid dynamic regime determined by the kinetic energy of gas separating from the kimberlite melt. Its main source is gas released during retrograde boiling of kimberlite magma during a drop in pressure in near-surface conditions. Participation of other gas phase sources is also possible: fracture and formation waters of host rocks, lacustrine and marine waters during an eruption into an aquatic environment. It is this fluid phase in a free form that leads to the pipe-shaped cavity development, and, acting as a transmagmatic flow of gas bubbles, promotes the transportation of solid phases, including diamonds, in the kimberlite magma. Based on the proposed kimberlite pipe formation mechanism, their morphological features will depend on the fluid dynamic regime of this process, which is determined by the following factors: the pressure of the magma gases at the moment of the beginning of their separation from the magma; the pressure difference from the pipe origin level to the Earth’s surface; the volume of the degassed part of the magmatic system (its fluid potential); the depth of the beginning of magma boiling; the explosive stage duration; the continuity or discreteness of the explosive and intrusive processes; the duration of the interval between explosive acts; the spatial compatibility or disunity of individual explosive acts; participation of only magmatic fluid or additional fluid involvement from an external source; nature of rock permeability in the fluid passage interval; surface degassing environment (atmosphere or aquatic environment).
Magma degassing in a fracture-type intrusion during its ascent to the surface begins from the head of the magmatic column and only from the moment of direct connection of the kimberlite fluid magmatic system with the paleosurface. Degassing is quickly localized at the base of the future pipe in the area where the pressure drop is maximum. As the pressure drops, the degassing front shifts inward and expands, capturing ever larger magma volumes in which the fluid pressure has not yet dropped to the solubility limit of gas under the given thermodynamic parameters. This results in the emergence of a columnar region of magma degassing in the dike under the base of the forming pipe-shaped kimberlite body, within which a flow of gas bubbles will exist until the moment of degassing is complete (Fig.4).
When magma enters the cavity of a forming kimberlite pipe, diamond crystals together with other solid phases will move in the fluid flow upwards to the Earth’s surface with the participation of natural flotation. Consequently, the distribution of diamonds within a kimberlite pipe will be determined by the fluid dynamic regime of their formation, which is the fundamental basis for developing the diamond content criteria of kimberlite and lamproite bodies. The probability of an active fluid dynamic regime in dike-like bodies and sills is notably lower than in pipes, which significantly reduces their potential diamond content.
The magma degassing rate will be determined by the initial fluid phase pressure gradient values at the kimberlite pipe base and the fluid magmatic substance viscosity, which is functionally related to the kimberlite melt viscosity, its fluid saturation, and the amount of suspended solid phase. This mechanism, whose element is natural flotation, can explain many distribution features within kimberlite systems of mantle xenoliths, diamonds, and other solid phases, which are in the kimberlite melt during its intrusion.
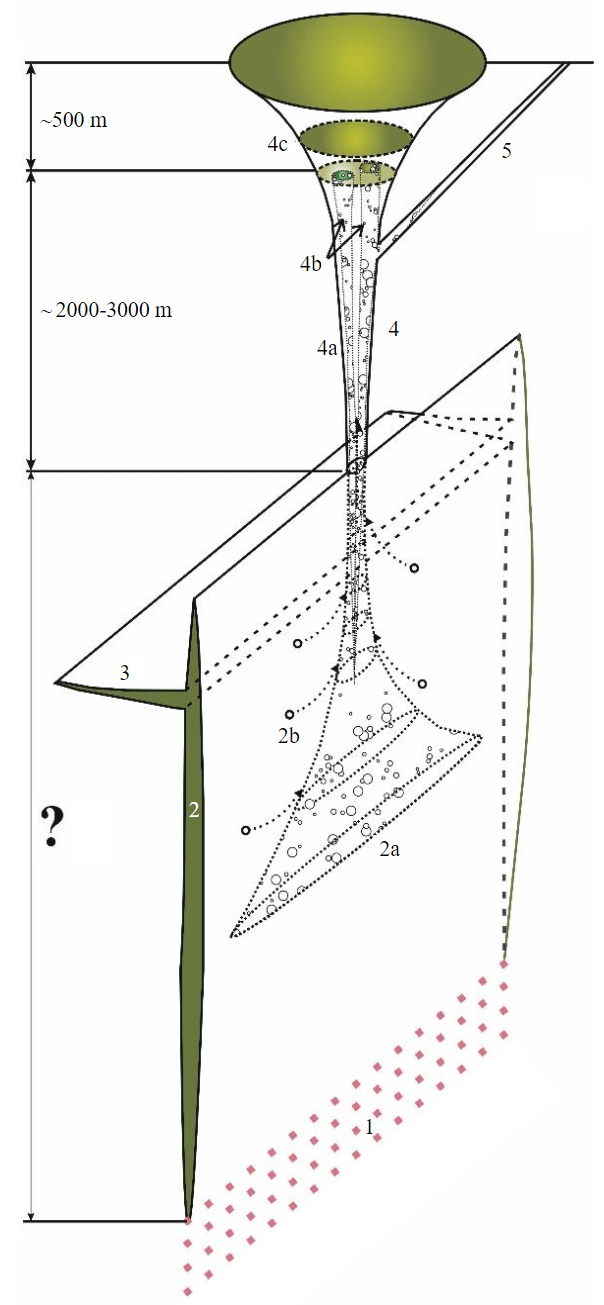
Fig.4. Diamond concentration in the near-surface kimberlite (lamproite) body
1 – relict dike; 2 – feeder dike (2a – core zone of active degassing; 2b – direction of gas component collection); 3 – kimberlite sill; 4 – kimberlite pipe (4a – diatreme part; 4b – transmagmatic fluid flows; 4c – crater part); 5 – outlet dike
It is important to emphasize that the active influence of fluid dynamics is great at the level of kimberlite and lamproite pipe formation. Analysis of fracturing in the rocks hosting kimberlite bodies led S.I.Kostrovitskii and other researchers to the conclusion about the pre-pipe nature of all fractures, the activation of which near kimberlite bodies is associated with a local stress field arising during the kimberlite melt intrusion [46-48]. The nature of the combination of fluid dynamics and tectonics is reflected in the morphological features of kimberlite bodies. The joint influence of tectonics and fluid dynamics is manifested in the combination of planar and conical elements in the kimberlite bodies morphology, reflecting the planar symmetry of tectonic and central symmetry of fluid dynamic processes, respectively. This is observed both in the morphological features of the cross sections of kimberlite bodies (Fig.5, a) and in their vertical section (Fig.5, b) with a change from predominantly linear forms to conical ones towards the surface, which is determined by an increase in the fluid flow rate during the kimberlite magma degassing as it moves toward the surface and the pressure gradient increases [48].
The nature of the intrusion of kimberlite magma transported in a limited portion to the near-surface zones (the first km from the paleo-surface) is determined by the fluid dynamic regime of the magmatic systems being developed. In conditions of relatively closed systems that have no direct connection with the Earth’s surface, mainly dikes and sills are formed. When a direct hydrodynamic connection of local kimberlite fluid magmatic systems with the Earth’s surface occurs, tubular bodies emerge in the frontal parts of magma-conducting fractures as a result of the heterogenization of the fluid-saturated kimberlite melt, in which the fluid phase begins to play a morphogenetic role and determines vertical and lateral differentiation of the heterophase fluid magmatic system. The outline of this process, which is accompanied by the emergence at the upper levels of two fundamentally different magmatic kimberlite systems in terms of fluid dynamics, is shown in Fig.6.
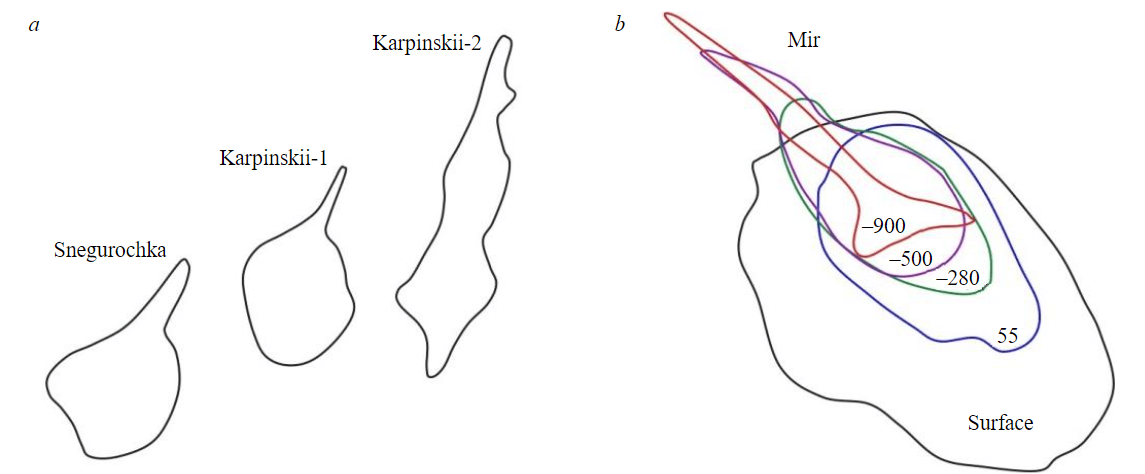
Fig.5. The ratio of linear and rounded elements in the kimberlite pipe cross-section on the surface (a) and at different hypsometric levels (b)
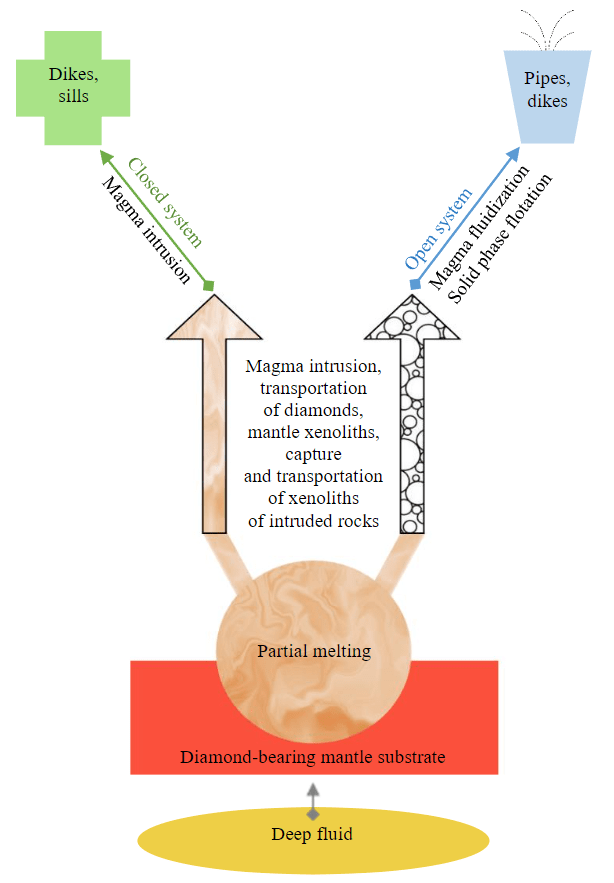
Fig.6. Development of diamond-bearing fluid magmatic system at different formation levels
Conclusion
The analysis of the proposed genetic geological model of the diamond-bearing fluid magmatic system allows us to draw the following conclusions:
- At mantle depths corresponding to the diamond formation levels, hydrogen, helium, carbon dioxide, nitrogen, methane, and heavy hydrocarbons are present in free and dissolved form in molten matter. The immediate environment for diamond crystallization may be iron-nickel or silicate-carbonate melts.
- The most efficient upward movement of diamond-bearing kimberlite magma is realized by the discrete-pulse mechanism of autonomous migration of the hosting fracture cavity, which ensures a high rate of magma ascent and long-term preservation of thermodynamic conditions for diamond stability in it. Depending on the starting parameters of the ascending magma portion, its movement to the surface can be carried out in a single or multiple act. The key parameters of the initial melt portion, determining its migration potential, are the volume of the melt, its temperature, pressure and the content of the dissolved gas phase. During the upward movement of the kimberlite-bearing fracture, the cured seam along its movement route can remain permeable for a long time, which makes it possible to replenish the ascending kimberlite melt portion with mantle fluid, which can cause resumption of fracture movement. During the upward ascent, a local fracture melt portion can capture liquid and solid matter fragments along the movement route, including diamonds formed at higher levels.
- Diamonds transported by the mechanism of autonomous migration of a fracture filled with fluidized melt, along with mantle xenoliths, are in a solid state and therefore can be captured when the lower part of the fracture collapses and trace its movement. These diamonds, due to being in a solid environment, can experience plastic and brittle deformations.
- Not only kimberlite (lamproite) pipes, dikes and sills can be diamond-bearing, but also other rocks that fell on the route of the ascending movement of a local portion of diamond-bearing fluid magmatic substance (crystalline basement rocks brought to the surface as a result of high-amplitude uplifts). This is the supposed primary source of diamonds in the Anabar placers, which has not yet been identified. A potential indicator of such local diamond-bearing structures may be chromium anomalies in felsic metamorphites that arose as a result of chrome-spinel (typical kimberlite minerals) grains capture along the route of a local portion of diamond-bearing fluidized magma movement.
- The upward movement of any deep fluids – silicate melts with gases dissolved in them, gases themselves (including hydrocarbons) – can be realized by the mechanism of autonomous discrete-pulse migration of fluid-filled fractures. The high rate of their upward movement allows transportation to the surface without significant losses as a result of dissolution in melts and sorption on the surface of mineral phases.
References
- Litvinenko V.S., Petrov E.I., Vasilevskaya D.V. et al. Assessment of the role of the state in the management of mineral resources. Journal of Mining Institute. 2023. Vol. 259, p. 95-111. DOI: 10.31897/PMI.2022.100
- Shirey S.B., Shigley J.E. Recent Advances in Understanding the Geology of Diamonds. Gems & Gemology. 2013. Vol. 49. N 4, p. 188-222. DOI: 10.5741/GEMS.49.4.188
- Kenney J.F., Kutcherov V.A., Bendeliani N.A, Alekseev V.A. The evolution of multicomponent systems at high pressures: The thermodynamic stability of the hydrogen-carbon system: The genesis of hydrocarbons and the origin of petroleum. Proceedings of the National Academy of Sciences. 2002. Vol. 99. N 17, p. 10976-10981. DOI: 10.1073/pnas.172376899
- Melton C.E., Giardini A.A. The Composition and Significance of Gas Released from Natural Diamonds from Africa and Brazil. American Mineralogist. 1974. Vol. 59. N 7-8, p. 775-782.
- Melton C.E., Giardini A.A. Experimental Results and a Theoretical Interpretation of Gaseous Inclusions Found in Arkansas Natural Diamonds. American Mineralogist. 1975. Vol. 60. N 5-6, p. 413-417.
- Popov O., Talovina I., Lieberwirth H., Duryagina A. Quantitative Microstructural Analysis and X-ray Computed Tomography of Ores and Rocks – Comparison of Results. Minerals. 2020. Vol. 10. Iss. 2. N 129. DOI: 10.3390/min10020129
- Smith E.M., Shirey S.B., Wuyi Wang. The Very Deep Origin of the World’s Biggest Diamonds. Gems and Gemology. 2017. Vol. 53. N 4, p. 388-403. DOI: 10.5741/GEMS.53.4.388
- Mukhina E., Kolesnikov A., Kutcherov V. The lower pT limit of deep hydrocarbon synthesis by CaCO3 aqueous reduction. Scientific Reports. 2017. Vol. 7. N 5749. DOI: 10.1038/s41598-017-06155-6
- Milashev V.A. Kimberlites and deep geology. Leningrad: Nedra, 1990, p. 167 (in Russian).
- Milashev V.A. Diatremes. Leningrad: Nedra, 1984, p. 268 (in Russian).
- Smith E.M., Shirey S.B., Nestola F. et al. Large gem diamonds from metallic liquid in Earth’s deep mantle. Science. 2016. Vol. 354. Iss. 6318, p. 1403-1405. DOI: 10.1126/science.aal1303
- Alekseev V.I., Marin Yu.B. Compositional Variations of Pyrochlore Group Minerals in Ongonite and Zwitter of the Verkhneurmiysky Pluton, Russian Far East. Geology of Ore Deposits. 2020. Vol. 61. N 7, p. 654-662. DOI: 10.1134/S107570151907002X
- Cashman K.V., Sparks R.S.J. How volcanoes work: A 25 year perspective. Geological Society of America Bulletin. 2013. Vol. 125. N 5-6, p. 664-690. DOI: 10.1130/B30720.1
- Butvina V.G. Possible diamondiferous melts. Research publications. 2014. N 11 (15), p. 48-54 (in Russian).
- Litvin Yu.A., Vasilev P.G., Bobrov A.V. et al. Parental Media of Natural Diamonds and Primary Mineral Inclusions in Them: Evidence from Physicochemical Experiment. Geochemistry International. 2012. Vol. 50. N 9, p. 726-759. DOI: 10.1134/S0016702912070051
- Vasilev E.A., Kozlov A.V. Hydrogen in diamond and a thermal history of diamond crystals. Proceedings of the Russian Mineralogical Society. 2018. Vol. 147. N 6, p. 103-112 (in Russian). DOI: 10.30695/zrmo/2018.1476.05
- Skuzovatov S.Yu., Zedgenizov D.A., Shatsky V.S. et al. Composition of cloudy microinclusions in octahedral diamonds from the Internatsionalnaya kimberlite pipe (Yakutia). Russian Geology and Geophysics. 2011. Vol. 52. № 1, p. 85-96. DOI: 10.1016/j.rgg.2010.12.007
- Litvin Yu.A. Physicochemical formation conditions of natural diamond deduced from experimental study of the eclogite-carbonatite-sulfide-diamond system. Geology of Ore Deposits. 2012. Vol. 54. N 6, 443-457. DOI: 10.1134/S1075701512060062
- Smith E.M., Wuyi Wang. Fluid CH4 and H2 trapped around metallic inclusions in HPHT synthetic diamond. Diamond and Related Materials. 2016. Vol. 68, p. 10-12. DOI: 10.1016/j.diamond.2016.05.010
- Frost D.J., Liebske C., Langenhorst F. et al. Experimental evidence for the existence of iron-rich metal in the Earth’s lower mantle. Nature. 2004. Vol. 428. N 6981, p. 409-412. DOI: 10.1038/nature02413
- Persikov E.S., Bukhtiyarov P.G., Aranovich L.Ya. et al. Experimental Modeling of Formation of Native Metals (Fe, Ni, Co) in the Earth’s Crust by the Interaction of Hydrogen with Basaltic Melts. Geochemistry International. 2019. Vol. 57. N 10, p. 1035-1044. DOI: 10.1134/S0016702919100082
- Klepikov I.V., Vasilev E.A., Antonov A.V. Regeneration Growth as One of the Principal Stages of Diamond Crystallogenesis. Minerals. 2022. Vol. 12. Iss. 3. N 327. DOI: 10.3390/min12030327
- Kostrovitskii S.I., Spetsius Z.V., Yakovlev D.A. et al. Atlas of primary diamond deposits of Yakutian kimberlite province. Mirnyi: ALROSA, 2015, p. 480 (in Russian).
- Stachel T., Aulbach S., Harris J.W. Mineral Inclusions in Lithospheric Diamonds. Reviews in Mineralogy and Geochemistry. 2022. Vol. 88. N 1, p. 307-391. DOI: 10.2138/rmg.2022.88.06
- Green B.L., Collins A.T., Breeding C.M. Diamond Spectroscopy, Defect Centers, Color, and Treatments. Reviews in Mineralogy and Geochemistry. 2022. Vol. 88. N 1, p. 637-688. DOI: 10.2138/rmg.2022.88.12
- Persikov E.S., Bukhtiyarov P.G., Polskoi S.F., Chekhmir A.S. Interaction of hydrogen with magmatic melts. Experiment in solving current geological issues. Moscow: Nauka, 1986, p. 48-70 (in Russian).
- Skublov S.G., Berezin A.V., Xian-Hua Li et al. Zircons from a Pegmatite Cutting Eclogite (Gridino, Belomorian Mobile Belt): U-Pb-O and Trace Element Constraints on Eclogite Metamorphism and Fluid Activity. Geosciences. 2020. Vol. 10. Iss. 5. N 197. DOI: 10.3390/geosciences10050197
- Sokol A.G., Khokhryakov A.F., Borzdov Yu.M. et al. Solubility of carbon and nitrogen in a sulfur-bearing iron melt: Constraints for siderophile behavior at upper mantle conditions. American Mineralogist. 2019. Vol. 104. Iss. 12, p. 1857-1865. DOI: 10.2138/am-2019-7103
- Mitchell R.H. Kimberlites. Mineralogy, Geochemistry, and Petrology. Springer, 1986, p. 442. DOI: 10.1007/978-1-4899-0568-0
- Palyanov Yu.N., Sokol A.G., Khokhryakov A.F., Kruk A.N. Conditions of diamond crystallization in kimberlite melt: Experimental data. Russian Geology and Geophysics. 2015. Vol. 56. N 1-2, p. 196-210. DOI: 10.1016/j.rgg.2015.01.013
- Melton C.E., Giardini A.A. The nature and significance of occluded fluids in three Indian diamonds. American Mineralogist. 1981. Vol. 66. N 7-8, p. 746-750.
- Sokol A.G., Tomilenko A.A., Bulbak T.A. et al. Composition of reduced mantle fluids: Evidence from modeling experiments and fluid inclusions in natural diamond. Russian Geology and Geophysics. 2020. Vol. 61. N 5-6, p. 663-674. DOI: 10.15372/RGG2020103
- Peña-Alvarez M., Brovarone A.V., Donnelly M.-E. et al. In-situ abiogenic methane synthesis from diamond and graphite under geologically relevant conditions. Nature Communications. 2021. Vol. 12. N 6387. DOI: 10.1038/s41467-021-26664-3
- Sharma A., Cody G.D., Hemley R.J. In Situ Diamond-Anvil Cell Observations of Methanogenesis at High Pressures and Energy and Fuels. 2009. Vol. 23. Iss. 11, p. 5571-5579. DOI: 10.1021/ef9006017
- Scott H.P., Hemley R.J., Mao H.-k. et al. Generation of methane in the Earth’s mantle: In situ high pressure-temperature measurements of carbonate reduction. Proceedings of the National Academy of Sciences. 2004. Vol. 101, p. 14023-14026. DOI: 10.1073/pnas.0405930101
- Sonin V.M., Bulbak T.A., Zhimulev E.I. et al. Synthesis of Heavy Hydrocarbons under P–T Conditions of the Earth’s Upper Mantle. Doklady Earth Sciences. 2014. Vol. 454. Part 1, p. 32-36. DOI: 10.1134/S1028334X1401005X
- Lobanov S.S., Pei-Nan Chen, Xiao-Jia Chen et al. Carbon precipitation from heavy hydrocarbon fluid in deep planetary interiors. Nature Communications. 2013. Vol. 4. N 2446. DOI: 10.1038/ncomms3446
- Russell J.K., Sparks R.S.J., Kavanagh J.L. Kimberlite Volcanology: Transport, Ascent, and Eruption. Elements. 2019. Vol. 15. N 6, p. 405-410. DOI: 10.2138/gselements.15.6.405
- Spera F.J. Carbon dioxide in petrogenesis III: role of volatiles in the ascent of alkaline magma with special reference to xenolith-bearing mafic lavas. Contributions to Mineralogy and Petrology. 1984. Vol. 88. Iss. 3, p. 217-232. DOI: 10.1007/BF00380167
- Smit K.V., D’Haenens-Johansson U.F.S., Howell D. et al. Deformation-related spectroscopic features in natural Type Ib-IaA diamonds from Zimmi (West African craton). Mineralogy and Petrology. 2018. Vol. 112. Suppl. 1, p. 243-257. DOI: 10.1007/s00710-018-0587-6
- Brown R.J., Manya S., Buisman I. et al. Eruption of kimberlite magmas: physical volcanology, geomorphology and age of the youngest kimberlitic volcanoes known on earth (the Upper Pleistocene/Holocene Igwisi Hills volcanoes, Tanzania). Bulletin of Volcanology. 2012. Vol. 74. Iss. 7, p. 1621-1643. DOI: 10.1007/s00445-012-0619-8
- Sparks R.S.J. Kimberlite Volcanism. Annual Review of Earth and Planetary Sciences. 2013. Vol. 41, p. 497-528. DOI: 10.1146/annurev-earth-042711-105252
- Brett R.C., Russell J.K., Andrews G.D.M. et al. The ascent of kimberlite: Insights from olivine. Earth and Planetary Science Letters. 2015. Vol. 424, p. 119-131. DOI: 10.1016/j.epsl.2015.05.024
- Wilson L., Head III J.W. An integrated model of kimberlite ascent and eruption. Nature. 2007. Vol. 447. N 7140, p. 53-57. DOI: 10.1038/nature05692
- Zhatnuev N.S., Vasiliev V.I., Sanzhiev G.D., Vasilieva Е.V. Model of kimberlite magmatism. Proceedings of the VI All-Russian Conference with International Participation “Ultramafic-mafic complexes: geology, structure, ore potential”, 19-20 September 2024, Sakhyurta, Russia. Irkutsk: Ottisk, 2019, p. 119-125 (in Russian).
- Kostrovitskii S.I. Physical conditions, hydraulics, and kinematics of kimberlite pipes filling. Novosibirsk: Nauka, 1976, p. 96 (in Russian).
- Kjarsgaard B.A. Kimberlite diamond deposits. Mineral Deposits of Canada: A Synthesis of Major Deposit Types, District Metallogeny, the Evolution of Geological Provinces and Exploration Methods. Geological Association of Canada, Mineral Deposits Division, 2007. Special Publication N 5, p. 245-272.
- Rezvukhina O.V., Skublov S.G. Rutile in diamondiferous metamorphic rocks: New insights from trace-element composition, mineral/fluid inclusions, and U-Pb ID-TIMS dating. Lithos. 2021. Vol. 394-395. N 106172. DOI: 10.1016/j.lithos.2021.106172